Abstract
In the present study, the relationship among iron-availability, antibacterial activity, role of meconium as an iron source and the activity of bacterial iron-uptake system (IUS) for bacterial growth in amniotic fluid (AF) were investigated. Staphylococcus aureus ATCC 6538 and its streptonigrin-resistant (SR) mutant with defective IUS were used as the test strains. The growth of S. aureus in AF was stimulated dosedependently by addition of meconium. Bacterial growth stimulated by meconium was re-inhibited dose-dependently by addition of iron-chelator, dipyridyl and apotransferrin. Iron concentration was correlated with the meconium content in AF (r2=0.989, p=0.001). High-affinity IUS of S. aureus was expressed only in AF but not in AF with meconium. The growth of SR strain was more retarded than that of the parental strain in the iron-deficient brain heart infusion (ID-BHI), clear AF and AF containing apotransferrin. The retarded growth of both strains in the ID-BHI and AF was recovered by addition of holotransferrin, hemoglobin and FeCl3. Taken together, the antibacterial activity of AF is closely related with low iron-availability. Bacterial growth in AF considerably depends on the activity of bacterial IUS. Meconium acts as one of the exogenous iron-sources and thus can stimulate bacterial growth in AF.
Normally clear amniotic fluid (AF) has antibacterial activity that reaches at peak in the 36-40 weeks of gestation (1, 2). This antibacterial activity is due to the combined effect of several antibacterial factors such as lysozyme, peroxidase, transferrin, lactoferrin, immunoglobulins, β-lysin, zinc and so on (3-6).
Meconium can stimulate bacterial growth in AF. So, meconium-stained AF (MSAF) is a very important risk factor of perinatal bacterial infections (7, 8). Several mechanisms have been proposed for meconium-associated perinatal infections, which include inhibition of antibacterial activity of AF, enhancement of bacterial growth and inhibition of phagocytosis and neutrophil oxidative burst (9-11). However, which component of meconium can stimulate bacterial growth in AF is not yet known. Instead, many researchers have thought meconium as a mere lump of nutrients to stimulate bacterial growth in AF (9, 12, 13).
Pathogenic bacteria require various kinds of nutrient to proliferate and cause infectious diseases in human body. However, bacterial growth is subjected to organic growth factors such as vitamins and trace elements such as iron because they function as cofactors of most enzymes inevitably required during metabolism, despite large amount of other nutrients being available. Actually some researchers reported that transferrin and lactoferrin could inhibit bacterial growth in AF by scavenging iron (5, 14-16).
Therefore, we tried to investigate the relationship between meconium and bacterial growth in AF focusing on iron that is one of the best-known trace elements and one of the bacterial growth factors. We used Staphylococcus aureus as the test bacterium because the bacterium had been known to have highly-developed high-affinity iron-uptake systems (IUS), both siderophore-mediated and staphylococcal transferrin-binding protein (StbA) or transferrin receptor-mediated IUS, thus could grow well even in iron-restricted harsh conditions (17-20).
AFs were obtained from the 70 pregnant women of the third trimester (30-36 weeks) for evaluation of fetal lung maturity by transabdominal amniocentesis. Among these, only the 25 clear AFs showing normal laboratory data and not containing any other contaminants such as blood and meconium were used for this study. The pooled AFs were prepared by mixing equal volume of the AFs. The pooled AF was filtered by disposable membrane filter (pore size 0.45 µm, Corning, NY, U.S.A.) for sterilization and removal of cells and stored at 4℃. Immediately before use, the AF was inactivated at 65℃ for 30 min. Self-defecated meconium was aseptically obtained within 24 hr after birth from the 10 neonates. MSAF was prepared as 10% (W/V) stock solution by mixing 1 g of each meconium with 100 mL of AF, and the stock solution was stored at 4℃. Working MSAF solution was prepared by diluting the stock solution whenever necessary. MSAF solution was centrifuged at 1,000 rpm to eliminate insoluble debris and to measure the iron concentration. Iron concentration of AF and MSAF was measured by Diagnostic Iron and Total Iron-Binding Capacity kit (Sigma Diagnostics, St. Louis, U.S.A.).
All laboratory glassware were immersed in 6N HCl solution for elimination of contaminated iron for more than two days, and washed ten times with deionized water (18.2 MΩ, Mili-Q plus, France) immediately before use. Iron-deficient (ID) medium was prepared from normal iron-sufficient (IS) Brain Heart Infusion broth (BHI; Difco Laboratories, Detroit, U.S.A.) according to the method described by Leong and Neilands (21). Residual iron concentrations of normal ISBHI and ID-BHI measured by Diagnostic Iron and Total Iron-Binding Capacity kit were 44.0 and 0.2 µg/dL, respectively. All reagents were purchased from Sigma Chemical Company (St. Louis, U.S.A.).
Streptonigrin, an aminoquinone antitumor agent, kills the bacteria with highly developed IUS, thus high intracellular iron-storage (22). So streptonigrin has been used for selection of a mutant with defective IUS. Streptonigrin-resistant (SR) strain was isolated from S. aureus ATCC 6538 by being exposed repeatedly to 125 ng of streptonigrin whose MIC was 1.95 ng (20). SR strain produced smaller amount of siderophore and expressed smaller amount of StbA on its cell wall in the iron-deficient brain heart infusion (ID-BHI) compared to the parental strain. The growth of SR strain was retarded in the ID-BHI but was not in the IS-BHI (Fig. 5).
About 1×106 cfu/mL of the bacteria grown in normal BHI broth was inoculated and cultured overnight in the BHI broth containing 0.2 mM dipyridyl, an iron chelator, to adapt the bacteria to the iron-restricted condition. About 1×105 cfu/mL of the bacteria grown in dipyridyl-BHI broth was inoculated into the ID-/IS-BHI and AF/MSAF, and shaking (200 rpm)-cultured at 37℃ for 12 or 24 hr. Bacterial growth was monitored by measuring optical density at 600 nm of wavelength of the culture supernatants withdrawn at appropriate intervals after inoculation.
Siderophore production was measured by a newly developed chrome azurol S (CAS) agar diffusion assay without modification (23). Siderophore production was shown as the square diameter of orange halo on the CAS agar plate.
Preparation of cell wall proteins was preformed according to the method described by Cheung and Fischetti (24). A 60 µg of cell wall proteins obtained from each culture was separated on 10% acrylamide gel at constant 20 mA, and then transferred onto nitrocellulose paper (Schleicher & Schuell, Keene, NH, U.S.A.). Receptor-ligand binding assay was performed according to the method described by Lim et al. (18). Horseradish peroxidase (HRP)-conjugated human transferrin (Jackson ImmunoResearch Laboratories, West Grove, PA, U.S.A.) was used as a probe. Diaminobenzidine (Sigma) was used as a substrate of HRP.
All results were from experiments repeated at least three times. Correlation between the iron concentration and the meconium content in AF was analyzed by Pearson correlation test and statistical analysis for comparison of siderophore production (mean±standard error) between the test groups was performed by Student t-test using the program Sigma-Stat version 1.0 by SPSS Science Inc. (Chicago, IL, U.S.A.).
Bacterial growth was inhibited in the clear AF, but stimulated dose-dependently in the MSAF containing 1, 5, 10 mg/mL of meconium (Fig. 1). The stimulated bacterial growth in the MSAF containing 5 mg/mL of meconium, which iron concentration was 80.5 µg/dL, was re-inhibited dose-dependently by dipyridyl, an iron-chelator (Fig. 2A) and apotransferrin (Fig. 2B).
To measure directly the iron concentration in AF according to the addition of meconium, MSAF containing 10 mg/mL of meconium was diluted two-fold in clear AF and iron concentration was measured (Fig. 3). Iron concentration in MSAF was well correlated with amount of meconium added (Y=14.3X+9.0, p=0.001, r2=0.989). The calculated iron concentrations in MSAF containing 1, 5 and 10 mg/mL of meconium used in this study were 23.3, 80.5 and 152.0 µg/dL, respectively.
To investigate the iron-availability in the clear AF and MSAF, we tried to observe the expression of staphylococcal high-affinity IUS, siderophore production and StbA expression which were expressed only in the iron-restricted conditions, in the clear AF and MSAF. As shown in the Fig. 4A, siderophore production was related reciprocally to the iron concentration in the medium, although small amount of siderophore was still produced in the IS-BHI (p<0.05). Unlike siderophore production, the expression of StbA was not observed in the IS-BHI at all (Fig. 4B). The expression of StbA was also depended on the iron concentration in the medium (Fig. 4C). In the clear AF, siderophore production was not detected while the expression of StbA was observed (Fig. 4A, B). In contrast, both IUS were not expressed in the MSAF. Although we could not confirm whether siderophore was produced or not in the clear AF, or whether the amount of siderophore produced in the clear AF was below the detection limit of the CAS agar diffusion assay, we could confirm that the iron-availability of AF was so low that StbA could be expressed while that of the MSAF was so high that the expression of StbA could be repressed.
Both strains, the parental strain with higher IUS activity and the SR strain with lower IUS activity, grew more actively in the IS-BHI of which iron concentration was 44.0 µg/dL than in the ID-BHI of which iron concentration was 0.2 µg/dL. When the bacteria were cultured in the iron-sufficient BHI, there was no difference in growth between the two strains (Fig. 5). However, when the bacteria were cultured in the ID-BHI, a remarkable difference of growth was observed between the two strains (Fig. 5A, 6C). The growth of the SR strain with lower IUS activity was more retarded in the IDBHI compared to that of the parental strain with higher IUS activity. The growth of both strains was retarded more severely in the ID-BHI with apotransferrin than only in the IDBHI (Fig. 6A). On the other hand, the growth of both strains was recovered in the ID-BHI with FeCl3, an inorganic iron-source, and hemoglobin and holotransferrin, organic iron-sources (Fig. 6B, D and E).
Bacterial growth in the clear AF according to addition of various iron sources was similar results to that in the ID-BHI. When the bacteria were cultured in the clear AF or AF containing apotransferrin, a remarkable difference in growth was observed between the two strains (Fig. 7A, C). The growth of the SR strain with lower IUS activity was more inhibited in the AF compared to that of the parental strain with higher IUS activity. On the other hand, the retarded growth of both the strains was recovered in the AF with FeCl3 and hemoglobin and holotransferrin (Fig. 7B, D and E). When the bacteria were cultured in the AF containing FeCl3 and hemoglobin, there was no difference in growth between the two strains. However, one noticeable point that is different from the results in the ID-BHI was observed (Fig. 7B). When the bacteria were cultured in the AF containing holotransferrin, the growth of the SR strain was less active than that of the parental strain unlike those in the ID-BHI.
Iron is the fourth abundant metal on earth and one of the trace elements inevitably required for survival and proliferation of all living things including microorganisms. Most extracellular iron found in body fluids such as plasma, secretions and AF is bound to the high-affinity iron-binding glycoproteins such as transferrin and lactoferrin, resulting in little free iron being available. Iron is an essential element in a number of key enzyme systems in most bacterial species and yet the levels of freely available iron within human body are too low to sustain growth (25). So, the restricted iron-availability itself is one of the natural immune defense mechanisms. On the other hand, many bacteria respond to the environmental cue of restricted iron-availability by de-repressing high-affinity IUS. The ability to express such IUS itself is a determinant of bacterial virulence (26).
To respond to the restricted iron-availability, most bacteria express either siderophore-mediated IUS or transferrin receptor-mediated IUS. Particularly, S. aureus is known to express both IUS and grow actively in the severely iron-restricted conditions such as body fluids, compared with other pathogenic bacteria (17-20). Actually, we observed that S. aureus could grow in the severely iron-restricted condition in which other pathogenic bacteria including Escherichia coli could not grow (data not shown). This was the reason that S. aureus was used in this study although it was not a main causative agent in perinatal infections.
In this study, AF was a severely iron-restricted condition in which S. aureus could express StbA, a high-affinity IUS which is expressed only in the iron-restricted condition, but could not grow actively. This antimicrobial activity of AF was decreased by adding various iron sources including holotransferrin, but increased by adding apotransferrin. These results indicated that 'low iron-availability' itself was an antimicrobial factor in AF. In this study, siderophore production could not be detected by CAS agar diffusion assay (23). We could not verify whether siderophore was produced in AF, or whether siderophore production in AF was below the detection limit of CAS agar diffusion assay, or whether the detection of siderophore was interfered by ingredients of AF. To verify the production of siderophore in AF, the further consecutive study using more sensitive and stable method for measurement of siderophore is necessary.
Our results were supported by other researchers' results. Oka et al. (5) reported that the growth of Escherichia coli was inhibited in AF with apotransferrin, but stimulated in AF with holotransferrin. According to the results of Thadepalli and his colleagues (16), AF could be largely divided into the two categories, inhibitory AF and non-inhibitory AF. In the inhibitory AF, iron concentration was low, apotransferrin that do not bind to iron was elevated, and finally iron-saturation of transferrin was around 30%. On the other hand, in the non-inhibitory AF, iron concentration was elevated, apotransferrin was decreased, and finally iron-saturation of transferrin was more than 50%. This categorization was based on the fact that the higher iron-saturation of transferrin is, the easier bacteria can capture iron from transferrin (27).
Meconium-staining in AF stimulates bacterial growth. Therefore meconium staining in AF is a very important risk factor of perinatal bacterial infections (7, 8). Several mechanisms have been proposed for meconium-associated perinatal infections, which include inhibition of antibacterial activity of AF, enhancement of bacterial growth and inhibition of phagocytosis and neutrophil oxidative burst (9-11). However, which component of meconium stimulates bacterial growth in AF is not known yet. Many researchers have thought meconium as a mere lump of nutrients to stimulate nonspecifically bacterial growth when added in AF (9, 12, 13). Actually AF itself without meconium is also a highly nutritious condition, because when AF was heated at 100℃, its antibacterial activity was completely lost and bacterial growth was stimulated (5). In other words, the reason why bacteria can not grow in AF is not due to deficiency of nutrient.
Most pathogenic bacteria require various kinds of nutrient to proliferate and cause infectious diseases in human body. However, bacterial growth is subjected to organic growth factors such as vitamins and trace elements such as iron because they function as cofactors for most enzymes inevitably required during metabolism, despite large amount of other nutrient being available. It has been well known that meconium contains various trace elements including Fe, Cu, Mn, and so on (13).
So in this study, we tried to investigate the relationship between meconium-staining and bacterial growth in AF focusing on iron that is one of the best-known trace elements and one of the bacterial growth factors. According to our results, iron concentration in MSAF was proportional to the amount of meconium, bacterial growth was stimulated dose-dependently by meconium, the expression of StbA was repressed in MSAF, and the stimulated bacterial growth by meconium was re-inhibited dose-dependently by dipyridyl, an iron-chelator. These results indicated that meconium acted as an iron-source for bacterial growth in AF. If AF was stained by meconium which acts as an iron source, apotransferrin in AF was decreased, thus bacteria could capture easily iron from transferrin for growth as explained above (27).
Whether bacteria can grow in AF or not is depended on the activity of bacterial IUS as well as the presence or absence of antimicrobial factors. Bacteria with highly developed IUS can grow more actively in AF than bacteria with defective IUS. Thus bacterial IUS activity itself is known to be a virulence-determinant (25-27). So in this study, we compared the growth in AF between the parental strain with high IUS activity and the SR strain with defective IUS activity. SR strain expressed smaller amount of StbA on their cell wall and produced smaller amount of siderophore in the ID-BHI compared with the parental strain. The SR strain grew less actively than the parental strain in AF only or AF with apotransferrin and holotransferrin, but there was no difference in growth between the two strains in AF with FeCl3 and hemoglobin. These results indicated that the SR strain with defective IUS activity captured less efficiently iron from transferrin than the parental strain in AF. These results in AF were similar to those in the ID-BHI except for the fact that there was no difference in growth between the two strains in IDBHI with holotransferrin. We thought that the reason why there was some difference in growth between the two strains in AF with holotransferrin was due to the pre-existing apotransferrin in AF. Before adding holotransferrin into AF, considerable amount of apotransferrin was already contained in AF like other body fluids. It is well known that iron-saturation of transferrin in normal AF is also about 30%, and about 70% of transferrin exists in unbound form like in other body fluids. Accordingly, although holotransferrin was added in AF as in this study, the actual iron-saturation of transferrin in AF and the iron-availability was thought to be lower than being expected. However, this phenomenon was not happened in ID-BHI. Considering there was no difference in growth between the two strains in AF with FeCl3 and hemoglobin, the IUS activity was thought to play more important role in bacterial growth in AF with low iron-availability not containing any exogenous iron sources than in AF with high iron-availability
In conclusion, these results indicate that antibacterial activity of AF is closely related with the low iron-availability, bacterial growth in AF considerably depends on the activity of bacterial IUS, and meconium acts as one of the exogenous iron-sources for bacterial growth in AF. However, this study has a limitation that S. aureus is not a major causative agent of perinatal infections associated with MSAF. So, the further consecutive studies using major pathogens of perinatal infections are necessary.
Figures and Tables
Fig. 1
Dose-dependent growth stimulation by meconium in amniotic fluid (AF). About 1×105 cfu/mL of the preconditioned S. aureus ATCC 6538 was inoculated into the iron-sufficient BHI (IS-BHI) and AF containing 1, 5 and 10 mg/mL of meconium, respectively, and cultured with vigorous shaking (200 rpm) at 37℃ for 12 hr. At the indicated intervals, bacterial growth was measured by optical density at 600 nm of wavelength. Experiments were carried out at least three times with similar results, and a representative one is shown.

Fig. 2
Dose-dependent growth inhibition by dipyridyl (A) and apotransferrin (AT; B) in meconium-stained AF (MSAF; 5 mg/mL of meconium). About 1×105 cfu/mL of the preconditioned S. aureus ATCC 6538 was inoculated into the MSAF containing 10, 100 and 1,000 µg/mL of dipyridyl, and the MSAF containing 0.1 and 0.3 mg/mL of apotransferrin, respectively, and cultured with vigorous shaking (200 rpm) at 37℃ for 12 hr. At the indicated intervals, bacterial growth was measured by optical density at 600 nm of wavelength. Experiments were carried out at least three times with similar results, and a representative one is shown.

Fig. 3
Correlation between iron concentration and meconium content in amniotic fluid (AF). Iron concentration was well correlated with meconium content in AF [Y=14.33X+9.0 (r2=0.989, p=0.001)].

Fig. 4
(A) Siderophore production in the amniotic fluid (AF) and meconium-stained AF (MSAF; 5 mg of meconium). The squared diameter (mm2) indicates the production of siderophore (mean±standard error) in the culture supernatants. Symbol (#) indicates the results showing statistically-significant difference (p<0.05) between the ID- and IS-BHI. (B) Expression of staphylococcal transferrin-binding protein (StbA; about 38 kDa) in AF and MSAF, and (C) iron-dependent expression of StbA in ID-BHI with various concentrations of FeCl3. About 1×105 cfu/mL of the preconditioned S. aureus ATCC 6538 was inoculated into the test media. Bacterial growth was shown in Fig. 1, 5. Cell wall proteins were isolated from the bacterial pellets after 24 hr-cultures. A 60 µg of cell wall proteins was electrophoresed and transferred to nitrocellulose membrane. StbA was probed with human transferrin-conjugated with horseradish peroxidase. Abbreviations: ID-BHI for iron-deficient BHI, IS-BHI for iron-sufficient BHI, AF for amniotic fluid and MSAF for meconium-stained amniotic fluid.
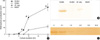
Fig. 5
Comparison of growth (A), siderophore production (B) and expression of transferrin-binding protein (StbA; C) between the parental strain (ATCC 6538) and its mutant (SR). About 1×105 cfu/mL of the preconditioned S. aureus ATCC 6538 was inoculated into the iron-deficient (ID) and the iron-sufficient (IS) BHI. Bacterial growth was measured by optical density at 600 nm of wavelength. The experiment was carried out at least three times with similar results, and a representative one was shown. The squared diameter (mm2) indicates the production of siderophore (mean±standard error) in the culture supernatants after 24 hr-cultures. Symbol (#) indicates the result showing statistically-significant difference (p<0.05) between the two strains. A 60 µg of cell wall proteins obtained from the bacterial pellets after 24 hr-culture was electrophoresed and transferred to nitrocellulose membrane. StbA was probed with human transferrin-conjugated with horseradish peroxidase.

Fig. 6
Growth according to the addition of various iron sources into the iron-deficient (ID) BHI. Two bacterial strains were used: S. aureus ATCC 6538 standard strain and streptonigrin-resistant (SR) strain. About 1×105 cfu/mL of the bacteria was inoculated into the ID-BHI (C) containing 0.5 mg/ mL of apotransferrin (A), 0.5 mg/mL of holotransferrin (B), 5 µM of FeCl3 (D) and 0.5 mg/mL of hemoglobin (E). Bacterial growth was measured by optical density (OD) at 600 nm of wavelength at the indicated intervals. Experiments were carried out at least three times with similar results, and a representative result was shown.

Fig. 7
Growth according to the addition of various iron sources into the amniotic fluid (AF). Two bacterial strains were used: S. aureus ATCC 6538 standard strain and streptonigrin-resistant (SR) strain. About 1×105 CFU/mL of the bacteria was inoculated into the AF (C) containing 0.5 mg/mL of apotransferrin (A), 0.5 mg/mL of holotransferrin (B), 5 µM of FeCl3 (D) and 0.5 mg/mL of hemoglobin (E). Bacterial growth was measured by optical density (OD) at 600 nm of wavelength at the indicated intervals. Experiments were carried out at least three times with similar results, and a representative result was shown.
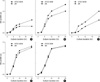
AKNOWLEDGEMENT
This study was supported by a grant (R01-2001-00120) from Korea Science and Engineering Foundation, and by Research Fund from Chosun University, 2003.
References
1. Abbasi IA, Hemming VG, Eglinton GS, Johnson TR. Proliferation of group B streptococci in human amniotic fluid in vitro. Am J Obstet Gynecol. 1987. 156:95–99.


2. Evans HE, Levy E, Glass L. Effect of amniotic fluid on bacterial growth. Obstet Gynecol. 1977. 49:35–37.
3. Galask RP, Snyder IS. Antimicrobial factors in amniotic fluid. Am J Obstet Gynecol. 1970. 106:59–65.


4. Honkonen E, Näntö V, Hyörä H, Vuorinen K, Erkkola R. Trace elements and antibacterial activity in amniotic fluid. Biol Neonate. 1986. 50:21–26.


5. Oka K, Hagio Y, Tetsuoh M, Kawano K, Hamada T, Kato T. The effect of transferrin and lysozyme on the antibacterial activity of amniotic fluid. Biol Res Pregnancy Perinatol. 1987. 8:1–6.
6. Schlievert P, Johnson W, Galask RP. Bacterial growth inhibition by amniotic fluid. VI. Evidence for a zinc-peptide antibacterial system. Am J Obstet Gynecol. 1976. 125:906–910.
7. Tran SH, Caughey AB, Musci TJ. Meconium-stained amniotic fluid is associated with puerperal infections. Am J Obstet Gynecol. 2003. 189:746–750.


8. Edwards RK. Meconium-stained amniotic fluid and its association with obstetric infections. Prim Care Update OB/Gyns. 1998. 5:315–318.


9. Romero R, Hanaoka S, Mazor M, Athanassiadis AP, Callahan R, Hsu YC, Avila C, Nores J, Jimenez C. Meconium-stained amniotic fluid: A risk factor for microbial invasion of the amniotic cavity. Am J Obstet Gynecol. 1991. 164:859–862.


10. Hoskins IA, Hemming VG, Johnson TR, Winkel CA. Effects of alterations of zinc-to-phosphorus ratios and meconium content on Group B Streptococcus growth in human amniotic fluid in vitro. Am J Obstet Gynecol. 1987. 157:770–773.


11. Clark P, Duff P. Inhibition of neutrophil oxidative burst and phagocytosis by meconium. Am J Obstet Gynecol. 1995. 173:1301–1305.


12. Florman AL, Teubner D. Enhancement of bacterial growth in amniotic fluid by meconium. Brief clinical and laboratory observations. J Pediatr. 1969. 74:111–114.
13. Friel JK, Matthew JD, Andrews WL, Skinner CT. Trace elements in meconium from preterm and full-term infants. Biol Neonate. 1989. 55:214–217.


14. Larsen B, Snyder IS, Galask RP. Transferrin concentration in human amniotic fluid. Am J Obstet Gynecol. 1973. 117:952–954.


15. Auger P, Marquis G, Dallaire L, Joly J. Stunted growth Candida albicans in human amniotic fluid in vitro. J Lab Clin Med. 1980. 95:272–281.
16. Thadepalli H, Gangopadhyay PK, Maidman JE. Amniotic fluid analysis for antimicrobial factors. Int J Gynaecol Obstet. 1982. 20:65–72.


17. Trivier D, Courcol RJ. Iron depletion and virulence in Staphylococcus aureus. FEMS Microbiol Lett. 1996. 141:117–127.
18. Lim Y, Shin SH, Lee SI, Kim IS, Rhee JH. Iron repressibility of siderophore and transferrin-binding protein in Staphylococcus aureus. FEMS Microbiol Lett. 1998. 163:19–24.
19. Taylor JM, Heinrichs DE. Transferrin binding in Staphylococcus aureus: involvement of a cell wall-anchored protein. Mol Microbiol. 2002. 43:1603–1614.


20. Chung JH, Park MH, Kim JH, Lim Y, Shin SH. Growth and siderophore production of staphylococci in human peritoneal dialysate. J Korean Med Sci. 2003. 18:158–162.


21. Leong SA, Neilands JB. Siderophore production by phytopathogenic microbial species. Arch Biochem Biophys. 1982. 218:351–359.


22. Yeowell HN, White JR. Iron requirement in the bactericidal mechanism of streptonigrin. Antimicrob Agents Chemother. 1982. 22:961–968.


23. Shin SH, Lim Y, Lee SE, Yang NW, Rhee JH. CAS agar diffusion assay for the measurement of siderophores in biological fluids. J Microbiol Methods. 2001. 44:89–95.


24. Cheung AL, Fischetti VA. Variation in the expression of cell wall proteins of Staphylococcus aureus grown on solid and liquid media. Infect Immun. 1988. 56:1061–1065.
26. Neilands JB. Siderophores: structure and function of microbial iron compounds. J Biol Chem. 1995. 270:26723–26726.
27. Simpson LM, Oliver JD. Ability of Vibrio vulnificus to obtain iron from transferrin and other iron-binding proteins. Curr Microbiol. 1987. 15:155–157.