Abstract
Background and Objectives
The overall purpose of this study was to investigate the role of cytochrome C oxidase (CcO) in preventing ischemia reperfusion-induced cardiac injury through gaseous signaling molecule pathways.
Materials and Methods
We used CcO inhibitor, potassium cyanide (KCN) to mimic the pre-treatment of gaseous signaling molecules in a global ischemia/reperfusion (IR) injury model in rats. Intracellular reactive oxygen species (ROS) was determined by measuring mitochondrial H2O2 and mitochondrial complex activity.
Results
KCN pre-treatment led to decreased infarction area after IR injury and improved cardiac function. KCN pre-treated group challenged with IR injury was associated with reduced ROS production through inhibition of activity and not downregulation of CcO expression. In addition, KCN pre-treatment was associated with enhanced expression and activity of mitochondrial antioxidase, suggesting the role of CcO in regulating IR injury through oxidative stress.
Cytochrome C oxidase (CcO) is a member of the oxidoreductase family. CcO is a large transmembrane protein complex that locates in the mitochondrion and the complex IV of respiratory electron transport chain. It is able to accept 4 electrons from cytochrome c and then transmit to one oxygen molecule to make two molecules of H2O, and consequently, adenosine triphosphate (ATP) is produced by ATP synthase.1) Therefore, CcO fine tunes the level of mitochondrial membrane potential, energy production, and reactive oxygen species (ROS) production.2) CcO is important for providing energy needed for metabolism; hence it is involved in different physiological and pathophysiological processes, including sepsis, stroke, encephalopathy, myopathy, motor neuron disease and cardiac dysfunction.3) The heart an important organ with high energy consumption, and reportedly, a major organ regulated by CcO activity, not only in humans with hypertrophic cardiomyopathy or fatal infantile cardioencephalomyopathy,4) but also under stress status like ischemia/reperfusion (IR) injury from animal studies.5) For instance, sustained ischemia is reportedly associated with protein kinase A-induced of CcO and leads to enhanced cardiac injury.6) On the other hand, ischemic preconditioning (IPC) could also phosphorylate CcO and inhibit its activity, which might be related to the protective role of IPC in cardiac function.7) The mechanistic role of CcO in signaling transduction involves the gaseous signaling molecule pathways including carbon monoxide (CO) and hydrogen sulfide (H2S) signal transduction.8) CO inhibits CcO, so that the ROS production is dramatically reduced and cardiac dysfunction is prevented.9) Collectively, previous studies suggest that CcO plays a role in cardiac tissue protection through neuronal nitric oxide synthase (nNOS).10) However, these are observational studies and the role of CcO in cardiac protection through gaseous signaling molecule pathways including NO, H2S and CO has not been defined.
NO, H2S and CO have high affinity with CcO and are able to inhibit the activity of CcO,11) hence, it is likely that CcO could be the common signaling target for gaseous signaling molecules. In the current study, we used potassium cyanide (KCN) to mimic the pre-treatment of gaseous signaling molecules, and investigate the role of CcO in preventing ischemia reperfusion-induced cardiac injury through gaseous signaling molecule pathways.
Sixty male Sprague-Dawley (SD) rats, weighted from 250g to 300 g, were used in the ex vivo Langendorff heart perfusion model, as the previously described IR injury model.12) Briefly, after heparin treatment (1000 IU/kg i.p.) and pentobarbital sodium anesthesia (60 mg/kg i.p.), the rat hearts were rapidly excised, cannulated, and immersed into cold Krebs Henseleit (K-H) solution of the following composition: NaCl 118.00 mmol/L, KCl: 4.75 mmol/L, CaCl2: 2.50 mmol/L, NaHCO3 24.80 mmol/L, MgCl2 · 6H2O 1.19 mmol/L, KH2PO4 1.19 mmol/L, glucose 11.1 mmol/L. Langendorff heart was perfused with oxygenated (95% O2/5% CO2, pH at 7.35-7.45 m, PO2 ≥500 mmHg and PCO2 37-45 mmHg) K-H solution at 37℃ at constant flow rate (4 mL/min) using constant flow Langendorff perfusion apparatus to ensure consistent perfusion over 10 min before the IR challenge. A saline-filled latex balloon was interposed into the left ventricle via the left atrium and linked to a pressure transducer to monitor the intraventricular pressure. Intraventricular pressure was monitored using Powerlab/sp8 system (ADInstruments Pty Ltd, Bella Vista, NSW, Australia) and pressure was adjusted using water sac to maintain left ventricle end diastolic pressure (LVEDP) between 4 to 10 mmHg. During the administration of ischemia-reperfusion, the heated chamber was plugged at the bottom and chamber was allowed to fill with effused buffer. When the heart was submerged in buffer, the flow of buffer into the heart was stopped to induce global ischemia. After 30 min of ischemia, the flow was restored to initiate reperfusion. Hearts were reperfused for 30 minutes before terminating the experiment.13)
SD rats were divided into four groups as follows. Control group: rats in this group received continuous perfusion of K-H solution for 50 minutes. Since studies have reported the protective role of H2S, CO or NO on ischemia reperfusion injury if pre-treated with these important gaseous signal molecules for 10-30 minutes, we decided to pretreat the heart with KCN for 20 minutes to mimic the effect of these gaseous signal molecules.12) KCN pre-treatment group: rats were first perfused with K-H solution for 10 minutes followed by the perfusion of KCN (10 µM, 60178 from Sigma) containing K-H solution for 20 minutes, and another 20 minutes of continuous K-H solution perfusion to wash out the KCN in cardiac tissue. After completion of the first 50 minutes of perfusion, 30 minutes of perfusion was conducted in half of the rats in the KCN and Control groups, respectively, comprising the Control+IR group and KCN+IR group. Brief experimental scheme was shown in Fig. 1.
After the corresponding treatments, hearts from rats in 4 groups were excised and cut into 7-10 pieces of tissue with 1mm in thickness. Tissues were stained for conventional infarct size determination via 2,3,5-triphenyl tetrazolium chloride (TTC) staining. Briefly, all tissues were placed in 1% TTC solution at 37℃ for 15 minutes until the vital cardiac tissue was stained a deep-red color, and the infarcted tissue a pale grey color. Variable and infarcted myocardium was analyzed using ImageJ for each slice. The infarction size was defined as the percentage of infarcted size of the heart in the overall heart tissue area for each slice. The average of the infarct size was defined as the infarct size of each heart; and average of the infarct size of each group with standard deviation values were shown in the Figures.13)14)
In isolated hearts, LVEDP and left ventricular systolic pressure (LVSP) were measured using the balloon inserted into the left ventricle, as mentioned above. The cardiac systolic function parameter left ventricular diastolic pressure (LVDP) was further calculated using LVSP and LVEDP. All parameters were recorded with BIOPAC system (BIOPAC Systems Inc., Goleta, CA, USA). The maximum of first derivative of left ventricular pressure (+dP/dtmax) was analyzed using analytic software (BSL v3.7.3; BIOPAC Systems Inc., Goleta, CA, USA).13)14)
Hearts were excised into small pieces in mitochondrial isolation solution at 4℃ (Trehalos 300 mmol/L, HEPES 10 mmol/L, KCl 10 mmol/L, EDTA 1 mmol/L and 1% BSA, pH 7.4) and homogenized. The solution was then centrifuged at 600 g for 10 minutes at 4℃ and supernatant was collected. The supernatant was further centrifuged at 3600 g for 15 minutes and pellet was collected. Mitochondrial isolation solution was then used to re-suspend the pellet and centrifuged at 1000 g for 5 minutes. The supernatant was further centrifuged at 5500 g for 10 minutes and the pellet was re-suspended in isolation solution.
To further demonstrate the relationship between KCN treatment and ROS production, we performed the ischemia-reperfusion model in another set of rats treated with different doses of KCN. H2O2 measurement was performed as a parameter of ROS production. Intracellular ROS was measured using Amplex® Red according to the manufacturer's protocol. Briefly, mitochondria were dissolved in 100 µM Amplex® Red solution (sucrose 250 mmol/L, MOPS 10 mmol/L, KH2PO4 5 mmol/L, Amplex® Red 0.05 mmol/L, horse radish peroxidase (HRP) 2 U/mL, superoxide dismutase (SOD) 0.2 U/mL, pH 7.4) and incubated in the dark for 30 minutes. Fluorescence was measured in a fluorescent plate reader (Molecular Devices, LLC, Sunnyvale, USA) at excitation of 540 nm and emission of 590 nm.16) The sample readout value was subtracted by the value from a negative control solution without mitochondrion. The absolute value was calculated by a standard curve with standard H2O2.
Mitochondrial complex activity was measured used a commercially available Mito Complex IV Activity Assay Kits kit (GenMed Scientifics Inc., Wilmington, DE, USA). The activities of xanthine oxidase (XOD) and antioxidants were measured using commercially available kits. The XOD and SOD activity assay kits were from Nanjing Jiancheng Bioengineering Institute (Nanjing, China). The catalase (CAT) and glutathione peroxidase x (GPx) activity assay kits were from Beyotime Co. (Nantong, China). These experiments were performed according to the manufacturer's instructions.
Mitochondria were collected and lysed, and the proteins were extracted and quantified using bicinchoninic acid assay (BCA) reagent (Shen Neng Bo Cai Corp., Shanghai, China). Protein samples were separated using 12.5% sodium dodecyl sulfate-polyacrylamide gel electrophoresis gels and then transferred to polyvinylidene fluoride (PVDF) membrane. Membranes were blocked with 5% non-fat milk for 2 hours at room temperature and then probed overnight with primary antibodies. After washing with tris-buffered saline with Tween 20 thrice for a total of 15 minutes, membranes were incubated with horseradish peroxidase-conjugated secondary antibodies for 2 hours at room temperature. The specific bands were detected by enhanced chemiluminescence substrate (Millipore, MA, USA).
Data were shown as mean±standard deviation. Statistical analysis was performed using SPSS 16.0 software (SPSS Inc., IBM Co., Chicago, IL, USA). Unpaired Student's t test was used to check the discrepancy between two groups. One-way analysis of variance (ANOVA) was used to test the differences when more than two groups were included, and a post-hoc analysis was further used to measure the p value between two specific groups. A value of p<0.05 was considered as statistically significant.
IR injury led to 35±6.4% infarction in cardiac tissue, and pre-treatment of KCN resulted in a 45.7% reduction of infarction area (19±3.8%, p<0.05 vs. C group) (Fig. 2A, B). Due to the significantly reduced infarction area, the cardiac function of heart pre-treated with KCN was improved, as reflected by both max dP/dt (Fig. 2C) and LVDP (Fig. 2D). Briefly, compared with max dP/dt level of control group (800±102 mmHg/sec), that of KCN-IR group increased to 1500±185 mmHg/sec (p<0.05 vs. C-IR group), although both groups had a significantly lower max dP/dt level than control (C) group. Similar pattern was also observed in LVDP, another parameter of cardiac dysfunction (Fig. 2D).
Analyses of mitochondrial complex activity showed that IR injury significantly reduced the activities of mitochondrial respiratory chain complexes including complex I, II, III and IV (Fig. 3A, C, D and E, between C and C-IR groups, all p<0.05). Pre-treatment of KCN led to significantly reduced activity of complex IV and CcO (Fig. 3A), but not complex I, II and III (Fig. 3C, D, and E between C and KCN groups). The reduced complex IV activity was not due to the decreased protein level in mitochondria of KCN pre-treated cardiac tissue, as shown by western blot (Fig. 3B). Besides, IR showed no change in CcO activity (p>0.05 vs. KCN group) or the protein levels in C-IR and KCN-IR groups (Fig. 3A, B).
Amplex® Red, a well-recognized methodology in measuring intracellular ROS level, was used to measure the H2O2. The results indicated that IR significantly accelerated the mitochondrial ROS production, consistent with reports from others. However, pretreating hearts with KCN led to significantly reduced ROS production level in cardiac mitochondrion (Fig 4A, B), as compared with those without KCN pre-treatment. Moreover, KCN showed a concentration-dependent inhibitory effect on ROS production, reaching a plateau at 15 uM (Fig 4C, D).
KCN pre-treatment increased the activities of mitochondrial antioxidases including superoxide dismutases 1 (SOD1) (Fig. 5B), superoxide dismutases 2 (SOD2) (Fig. 5C) and GPx (Fig. 5D), through increased protein levels of the enzymes, as measured by western blot (Fig. 6A-C). However, KCN pre-treatment did not result in enhanced activity of CAT (Fig. 5E), or inhibition of the xanthine oxidase activity (XOD) due to IR (Fig. 5A).
The major physiologic role of CcO is to participate in energy metabolism in mitochondria.14) CcO is able to transfer the electron from cytochrome c to oxygen molecule, producing energy for ATP synthesis. Therefore, CcO is an important enzyme participating in various physiological and pathophysiologic conditions. Since brain and heart are the two most important organs that require extensive energy supply, pathophysiological role of CcO is mostly reported in brain and heart diseases.15) Mechanistically, CcO has high affinity to gaseous signaling molecules including CO, NO and H2S;11) and therefore, CcO may be involved in the gaseous signaling molecule pathways, including CO and H2S pathways.8) In addition, these gaseous signaling molecules play a protective role in cardiac ischemia perfusion injury.13) Based on the findings above, we hypothesized that these molecules have a common mechanism in preventing cardiac dysfunction induced by IR injury through regulation of CcO. To investigate the role of CcO in the pathogenesis of IR injury, we pretreated the heart with KCN, a selective inhibitor of CcO, before challenge with IR injury.
In the current study, the myocardial infarction area was significantly reduced by the KCN treatment (Fig 1A, B). In addition, after challenge with IR injury, the cardiac dysfunction became less severe in KCN pre-treated heart, as compared with control heart, indicating a role of CcO in deteriorating cardiac function during the process of IR. Thus, ROS overproduction played a role in this process based on KCN (10 uM) pre-treatment-induced mitochondrial H2O2 production, indicating less ROS formation (Fig 2A, B). Consistent with our finding, emerging evidence supports the role of ROS overproduction,17) as the major mechanism of IR injury in the heart.18) Besides, KCN pre-treatment showed a dose-dependent reduction of ROS, with the greatest inhibitory effect at 15 uM (Fig 2C, D), indicating a possible association between KCN and ROS production. Lesnefsky et al and Chen et al both reported that using the respiratory electron transport chain inhibitor could reduce the IR injury, consistent with our finding.19) However, our study has provided a more detailed dose effect of KCN on cardiac dysfunction protection under the condition of IR.
In addition, our study showed that the reduced ROS production and protection from cardiac dysfunction on IR challenge following KCN pre-treatment was associated with decreased CcO enzyme activity of (Fig. 3A). Western blot showed no significant decrease of CcO protein level before and after KCN treatment, or with and without IR challenge, suggesting a direct effect of KCN on regulation of CcO activity, rather than protein expression level. To further confirm the specificity of KCN on CcO activity in IR injury model, we measured other complexes in respiratory electron transport chain including complex I, II and III. No difference in the activity of these complexes was observed. Therefore, the role of KCN in protecting from cardiac injury during IR and reduced ROS production was through regulation of CcO activity rather than other enzymes in mitochondrial respiratory chain. Collectively, our data suggested the association between IR and CcO activity, but not CcO protein levels.
In addition to the mitochondrial complexes in respiratory chain to produce ROS after IR injury, XOD in the mitochondrial matrix was also able to produce ROS.20) Our data suggested that KCN pre-treatment did not influence the XOD activity or reduce the enhanced XOD activity upon IR injury challenge (Fig. 4). This suggested a negative role of KCN in regulating XOD, via regulating anti-oxidase enzyme activity in mitochondria. Our data suggested that this was the case because the activity of multiple anti-oxidase including SOD1, SOD2 and GPx were all elevated after KCN pre-treatment (Fig. 5). Based on our data, we propose that KCN pre-treatment led to elevated anti-oxidase activity in mitochondria, subsequently enhancing the antioxidant capacity of the mitochondrion. Excessive ROS production under IR injury challenge increased the ROS clearance rate by the enhanced antioxidant capacity of mitochondria, which mediated the protective role of KCN in IR injury of the heart. Studies have shown that gaseous signaling molecules H2S and CO could enhance the SOD activity and GPx expression level so as to protect cardiac function. Since KCN pre-treatment could increase the endogenous expression of anti-oxidase, which was similar to the mechanism of H2S and CO, it is likely that regulating CcO activity was the common protective mechanism for both H2S and CO.21)
In conclusion, KCN pre-treatment reduced the severity of IR injury. The potential mechanism included increased endogenous anti-oxidase activity and consequently, enhanced clearance of ROS.
Figures and Tables
Fig. 1
Experimental scheme. K-S: Krebs Henseleit, C: control group, KCN: potassium cyanide pre-treatment group, C-IR: control+ischemia reperfusion group, KCN-IR: potassium cyanide pre-treatment+ischemia reperfusion group.
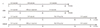
Fig. 2
Pre-treatment of IR resulted in decreased infarction area and improved cardiac function. (A, B) Myocardium infarct size (n=8) with and without KCN (10 uM) pre-treatment including representative images (A) and quantification (B). (C,D) Cardiac function of heart pre-treatment with and without KCN reflected by (C) max dP/dt and (D) LVDP (n=8). C: control group, KCN: potassium cyanide pre-treatment group, TTC: triphenyl tetrazolium chloride, C-IR: control+ischemia reperfusion group, KCN-IR: potassium cyanide pre-treatment+ischemia reperfusion group, LVDP: left ventricular developed pressure, dP/dt: the rate of rise of left ventricular pressure. *p<0.05.
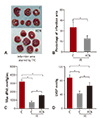
Fig. 3
KCN pre-treatment was associated with inhibited activity of CcO. Mitochondrial complex I, II, III, activity in cardiac tissue of rats challenged with and without IR injury in both control and KCN pre-treated groups. Cardiac (A) mitochondrial complex IV (n=6), (B) complex IV protein expression level (n=6), (C) complex I activity (n=6), (D) complex II activity (n=6) and (E) III (n=6) activity. C: control group, KCN: potassium cyanide pre-treatment group, VDAC1: voltage-dependent anion-selective channel 1, NADH: reduced form of nicotinamide-adenine dinucleotid, DCPIPH2: reduced form of dichlorophenalindophenol, QH2: reduced form of coenzyme Q10, C-IR: control+ischemia reperfusion group, KCN-IR: potassium cyanide pre-treatment+ischemia reperfusion group. *p<0.05.
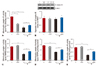
Fig. 4
KCN pre-treated group challenged with IR injury was associated with reduced ROS production. ROS production of cardiac tissue challenged with and without IR injury in both control and KCN pre-treated groups. H2O2 production by heart mitochondria oxidizing complex I (A) and (B) complex II substrates in the four groups. Effect of different concentrations of KCN pre-treatment (5, 10, 15 and 20 uM) on ROS production levels by complex I (C) and complex II (D) induced by IR treatment (both n=6). ROS: reactive oxygen species, C: control group, KCN: potassium cyanide pre-treatment group, C-IR: control+ischemia reperfusion group, KCN-IR: potassium cyanide pre-treatment+ischemia reperfusion group. *p<0.05.
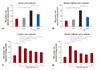
Fig. 5
Pre-treatment was associated with enhanced activity of mitochondrial antioxidase. ROS-related enzyme activity (XOD and antioxidase activities) in cardiac mitochondria of rats challenged with and without IR injury in both control and KCN pre-treated groups. The activity of (A) SOD1 (n=8), (B) SOD2 (n=8), (C) GPx (n=8), (D) CAT (n=8) and (E) XOD (n=8) in cardiac mitochondria. *p<0.05 between indicated groups. XOD: xanthine oxidase activity, SOD1: superoxide dismutases 1, SOD2: superoxide dismutases 2, GPx: glutathione peroxidase, CAT: catalase, C: control group, C: control group, KCN: potassium cyanide pre-treatment group, C-IR: control+ischemia reperfusion group, KCN-IR: potassium cyanide pre-treatment+ischemia reperfusion group.
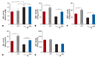
Fig. 6
Pre-treatment was associated with increased expression levels of mitochondrial antioxidase. ROS-related enzyme expression levels. The expression of antioxidase including (A) SOD1 (n=3), (B) SOD2 (n=3) and (C) GPx1(n=3) in cardiac mitochondria of rats challenged with and without IR injury in both control and KCN pre-treated groups. SOD1: superoxide dismutases 1, SOD2: superoxide dismutases 2, GPx: glutathione peroxidase, VDAC1: voltage dependent anion channel 1, C: control group, KCN: potassium cyanide pre-treatment group, C-IR: control+ischemia reperfusion group, KCN-IR: potassium cyanide pre-treatment+ischemia reperfusion group. *p<0.05.

Acknowledgments
This study was supported by National Natural Science Foundation of China (grant no. 81370254) and Research Foundation of the Health and Family Planning Commision of Hunna Province (grant no. 132015-028).
References
1. Tsukihara T, Aoyama H, Yamashita E, et al. Structures of metal sites of oxidized bovine heart cytochrome c oxidase at 2.8 A. Science. 1995; 269:1069–1074.
2. Hüttemann M, Helling S, Sanderson TH, et al. Regulation of mitochondrial respiration and apoptosis through cell signaling: cytochrome c oxidase and cytochrome c in ischemia/reperfusion injury and inflammation. Biochim Biophys Acta. 2012; 1817:598–609.
3. Groening P, Huang Z, La Gamma EF, Levy RJ. Glutamine restores myocardial cytochrome C oxidase activity and improves cardiac function during experimental sepsis. JPEN J Parenter Enteral Nutr. 2011; 35:249–254.
4. Bruno C, Martinuzzi A, Tang Y, et al. A stop-codon mutation in the human mtDNA cytochrome c oxidase I gene disrupts the functional structure of complex IV. Am J Hum Genet. 1999; 65:611–620.
5. Comi GP, Bordoni A, Salani S, et al. Cytochrome c oxidase subunit I microdeletion in a patient with motor neuron disease. Ann Neurol. 1998; 43:110–116.
6. Muller-Hocker J. Cytochrome-c-oxidase deficient cardiomyocytes in the human heart--an age-related phenomenon. A histochemical ultracytochemical study. Am J Pathol. 1989; 134:1167–1173.
7. Antonicka H, Mattman A, Carlson CG, et al. Mutations in COX15 produce a defect in the mitochondrial heme biosynthetic pathway, causing early-onset fatal hypertrophic cardiomyopathy. Am J Hum Genet. 2003; 72:101–114.
8. Papadopoulou LC, Sue CM, Davidson MM, et al. Fatal infantile cardioencephalomyopathy with COX deficiency and mutations in SCO2, a COX assembly gene. Nat Genet. 1999; 23:333–337.
9. Prabu SK, Anandatheerthavarada HK, Raza H, Srinivasan S, Spear JF, Avadhani NG. Protein kinase A-mediated phosphorylation modulates cytochrome c oxidase function and augments hypoxia and myocardial ischemia-related injury. J Biol Chem. 2006; 281:2061–2070.
10. Booth EA, Flint RR, Lucas KL, Knittel AK, Lucchesi BR. Estrogen protects the heart from ischemia-reperfusion injury via COX-2-derived PGI2. J Cardiovasc Pharmacol. 2008; 52:228–235.
11. Vogt S, Ramzan R, Weber P, et al. Ischemic preconditioning results in an ATP-dependent inhibition of cytochrome C oxidase. Shock. 2013; 40:407–413.
12. Sun WH, Liu F, Chen Y, Zhu YC. Hydrogen sulfide decreases the levels of ROS by inhibiting mitochondrial complex IV and increasing SOD activities in cardiomyocytes under ischemia/reperfusion. Biochem Biophys Res Commun. 2012; 421:164–169.
13. Whittington HJ, Hall AR, McLaughlin CP, Hausenloy DJ, Yellon DM, Mocanu MM. Chronic metformin associated cardioprotection against infarction: not just a glucose lowering phenomenon. Cardiovasc Drugs Ther. 2013; 27:5–16.
14. Burkard N, Williams T, Czolbe M, et al. Conditional overexpression of neuronal nitric oxide synthase is cardioprotective in ischemia/reperfusion. Circulation. 2010; 122:1588–1603.
15. Guo W, Cheng ZY, Zhu YZ. Hydrogen sulfide and translational medicine. Acta Pharmacol Sin. 2013; 34:1284–1291.
16. Zuckerbraun BS, Chin BY, Bilban M, et al. Carbon monoxide signals via inhibition of cytochrome c oxidase and generation of mitochondrial reactive oxygen species. FASEB J. 2007; 21:1099–1106.
17. Jain M, Rivera S, Monclus EA, et al. Mitochondrial reactive oxygen species regulate transforming growth factor-beta signaling. J Biol Chem. 2013; 288:770–777.
18. Diaz F. Cytochrome c oxidase deficiency: patients and animal models. Biochim Biophys Acta. 2010; 1802:100–110.
19. Nicholson CK, Calvert JW. Hydrogen sulfide and ischemia-reperfusion injury. Pharmacol Res. 2010; 62:289–297.
20. Chen Q, Camara AK, Stowe DF, Hoppel CL, Lesnefsky EJ. Modulation of electron transport protects cardiac mitochondria and decreases myocardial injury during ischemia and reperfusion. Am J Physiol Cell Physiol. 2007; 292:C137–C147.
21. Tanaka-Esposito C, Chen Q, Lesnefsky EJ. Blockade of electron transport before ischemia protects mitochondria and decreases myocardial injury during reperfusion in aged rat hearts. Transl Res. 2012; 160:207–216.