Abstract
Incomplete development and severe malformation of the heart result in miscarriage of embryos because of its malfunction as a pump for circulation. During cardiogenesis, development of the heart is precisely coordinated by the genetically-primed program that is revealed by the sequential expression of transcription factors. It is important to investigate how spatial allocation of the heart containing cardiomyocytes and other mesoderm-derived cells is determined. In addition, the molecular mechanism underlying cardiomyocyte differentiation still remains elusive. The location of ectoderm-, mesoderm-, and endoderm-derived organs is determined by their initial allocation and subsequent mutual cell-cell interactions or paracrine-based regulation. In the present work, we provide an overview of cardiac development controlled by the germ layers and discuss the points that should be uncovered in future for understanding cardiogenesis.
Defects in cardiac development become the causes of miscarriage. More than 80% of early abortion is thought to be ascribed to the chromosome abnormality. Among the total pregnancy, 12-15% ends in miscarriage.1)2) However, even after the heart beat is clinically recognized, some embryos do not develop due to unknown causes. Severe congenital heart diseases (CHDs) might be the cause of cessation of pregnancy. One percent of new babies are estimated to have CHDs.3)4) Mutations of transcription factors including Nkx2.5, Tbx5, GATA family transcription factors, and MEF2, which regulate cardiogenesis, result in CHDs (atrial septal defect, ventricular septal defect, tetralogy of Fallot, double outlets of right ventricle, and transposition of great arteries).5)6) Besides the regulation of genetic program-dependent expression of transcription factors for mesodermal cells that give rise to cardiomyocytes, the defects in the connection of great vessels that also originate from the mesoderm along with the heart result in CHDs.7) These abnormal developments of the hearts and the vessels are thought to be ascribed to the abnormal left-right axis formation during early embryogenesis.
Zebrafish is a useful model to study embryogenesis, because the embryos of zebrafish are transparent, can be manipulated easily to delete genes of interests and introduce transgenes, and grow extra-maternally.8)9)10)11) Early cardiogenesis can be monitored by cardiomyocyte-specific promoter-dependent fluorescent protein expression. Zebrafish has single atrium and single ventricle, while mouse and human have four chambers. Although this structural difference is present between mammals and zebrafish, the most genetic transcriptional regulation is conserved in zebrafish and mammals.12)13) Therefore, the regions of promoter/enhancer of the transcription factors have been used for marking cardiomyocytes.14)15) In addition to visualizing cardiomyocytes, the endocardium consisting of endothelial cells is observed using endothelium-specific promoter-controlled fluorescent protein expression.16) Furthermore, beside these mesoderm-derived cells or precursor cells, endodermal cells can be simultaneously visualized using endoderm-specific promoter-regulated fluorescence. The imaging of the embryos using the combination of the distinct tissue- or organ-specific promoters and fluorescent proteins enables us to demonstrate how the endoderm and the mesoderm simultaneously develop with mutual effect on each other.
Initial body planning is determined mainly by three axes: rostral-caudal axis, dorsal-ventral axis, and left-right axis.17) Among them, the left-right axis determination defines the position of the heart. This left-right axis formation follows the Kupffer's vesicle (KV) formation that corresponds to the Node of mammals. The dorsal forerunner cells (DFCs) of the endoderm give rise to KV-forming cells.18)19) Subsequently, these cells become ciliated to promote unidirectional flow in the KV.20) Motile cilia rotate unidirectionally from right to left to induce unidirectional flow in the KV.21) This flow leads to the asymmetric expression of Nodal, Lefty, and Pitx2 in the left side of the embryo,22) which causes left-right asymmetric patterning, which determines the position and the fate of cardiac precursor cells (CPCs) present in the lateral plate mesoderm.
We introduce our recent findings about the CPC migration governed by the endodermal cells in zebrafish. Furthermore, we overview how left-right axis is determined for spatial patterning of the heart during early embryogenesis and focus on what will be clarified to understand left-right asymmetry of organ position.
Even at the blastula stage of zebrafish embryos, the localization of the cells that are going to differentiate into atrial and ventricular precursor cells is determined.23) This lineage tracing is performed by the caged fluorescein. By tracking the fluorescein-positive cells, the initially positioned cells are analyzed for their movement before and after gastrulation. These results suggest the regulatory mechanism underlying the specification and spatial positioning of CPCs during and after gastrulation. Retinoic acid, canonical Wnt, bone morphogenetic protein, Nodal, and fibroblast growth factor are reported to regulate the number of cardiomyocytes by directly acting on CPCs or by indirectly affecting the pattering of three germ layers and subsequent positioning of CPCs.24)25)26)27)28) These signaling patterns during blastula stage are nicely summarized by Schier and Talbot.17) The signaling molecules externally affecting CPCs determine the positioning of three germ layers that are required for the formation of dorsal-ventral and rostral-caudal axes. Apelin and its receptor (Agtrl1b)-mediated signal determines the anterior-posterior length during gastrulation, thereby affecting the positioning of the heart.29) Agtrl1b is also expressed in the CPCs in the lateral plate mesoderm. Therefore, Apelin might directly regulate the migration of CPCs towards the midline (as described below in “heart tube formation”). Another axis (left-right axis) determined by the KV-mediated left-right asymmetry governs the positioning of heart, because the CPCs are positioned in the bilateral anterior lateral plate mesoderm by the 6-9 somite stages (ss) when the KV is completely organized.
While axes are determined during the blastula stage and the gastrula stage, hierarchical transcription factor-dependent cardiomyocyte specification progresses in the mesoderm. Transcriptional activation in the other two layers might affect cardiogenesis from early stage (specification) to late stage (the migration of CPCs toward midline to form heart tube). A mutant of Gata5 (faust) exhibits endodermal defects that cause cardia bifida.30) That of Raldh2 (necklace) exhibits an increase in cardiomyocytes as well as spinal cord defects.31) The CPCs express Gata family transcription factors (Gata4, 5, and 6), Nkx2.5, Hand2, and Tbx5. However, it remains unclear how the expression of these transcriptional factors are precisely regulated during the blastula stage and the gastrula stage.
Left-right asymmetry is determined by several steps (Fig. 1). The unidirectional flow by counterclockwise rotation of cilia in the KV of zebrafish is essential for left-right axis formation. Therefore, the establishment of KV formed by DFCs is of importance.32) However, it remains unclear the manner in which two dozens of DFCs are selected among endodermal cells and how these cells move posteriorly.19) The requirement of endodermal cells is proven by mutant fish embryos in which sox32 is depleted.33) A sox32-depleted mutant (Casanova) lacks the KV. Moreover, the essential role of DFCs in forming the KV is clearly demonstrated by the experiment in which DFCs are ablated.32) The T-box transcription factor, no tail, the nodal co-receptor, and one-eyed pinhead are also essential for formation of the KV, although these are not specifically expressed in the endoderm,32)34) suggesting mutual regulation among the ectoderm, endoderm, and mesoderm to form the KV.
DFCs can be visualized by the transgenic fish embryos, Tg (sox17: green fluorescent protein [GFP]),35) in which GFP expression is promoted by sox17 promoter activated in the endoderm (Fig. 2). Therefore, Tg fish can be employed to examine migration and morphology of DFCs and to inspect the effect of depleting genes of interests. The KV is formed during early stages (4-6 ss). When the DFCs migrate, they need to become a cluster and move in a manner dependent on collective cell migration. Thus, cell-cell adhesion tightened by Cadherin-1 is essential for migration.32) In later stage, the KV must form a rosette-like structure with a lumen filled with fluid. The migration of DFCs and the subsequent formation of cluster leading to the KV are disturbed by the reduction or the depletion of FGF signal regulators including Fibroblast growth factor receptor-1a (Fgfr1a), Fgf8, and Canopy1.36)37)
The KV is a transient structure formed during embryogenesis to determine left-right asymmetry.21) Defects in ciliogenesis and disruption of the KV lead to abnormal left-right patterning, suggesting the essential role of ciliated cells in the KV (Fig. 3). The reduction in molecules constituting intraflagellar transport machinery of cilia: Ift57 (hippi), Ift88 (polaris), and left-right dynein related (Lrdr1), results in the defects of ciliogenesis and subsequent disruption of left-right patterning (Fig. 1). Cilia-dependent unidirectional flow causes the laterality of expression of Southpaw (Spaw), a nodal-related protein, Lefty2, and Pitx2 in the left side of lateral plate mesoderm. The expression of these Nodal inhibitors is regulated by positive feedback loop of the Spaw. On the other hand, in the right side, the expression of Charon increases to suppress the Spaw-dependent transcription by binding to the Spaw, since the flow starts in the KV. However, it still remains controversial as how the flow in the KV triggers and initiates the expression of these genes.
There exist at least two models to initiate the left-right asymmetry by a unidirectional flow: one is morphogen gradient; the other is two cilia model in the node of mouse. In the mouse node, cilia-mediated flow promotes morphogen gradient between the left and right sides. The flowing particles are suggested to bring morphogens preferentially to the left side.38) Sonic Hedgehog and retinoic acids are thought to be morphogens to be delivered by the flow. In the latter model, rotating motile cilia promotes the flow, while the immotile cilia on the perinodal crown cells sense the flow. This is hypothesized from the fact that the PKD2 in mutant mice is expressed in the cilia of perinodal crown cells thus exhibiting the left-right asymmetry of organ positioning.39) Therefore, PKD2-dependent sensing mechanism in cilia is a key determinant for the left-right asymmetry. In the KV, there are no clear data on morphogens. Further studies are required to examine whether the sensing cilia are present in the KV.
Calcium ion (Ca2+) increases in the left side of the KV as compared to the mouse node.40)41) In the zebrafish, a Ca2+ indicator, flash-pericam, can monitor the increase in Ca2+ in the KV. Ca2+ increases in the left side at the 4-6 ss via a 1,3,4,5,6-pentakisphosphate 2-kinase,41) although how this inositol phosphatase activity is regulated in the KV. In addition, Ca2+ oscillation in the cilia is thought to regulate left-right asymmetry.42)43)44) Although the cilia of the KV are motile,20) they are proposed to express PKD2 and sense extracellular Ca2+, as the immotile cilia in mouse perinodal crown cells do, although the cilia have 9+2 type microtubules in the KV.4) The movement of cilia and intracellular Ca2+ can be monitored by the small GTPase, Arl13b, tagged with genetically encoded Ca2+ indicators, GCaMP5 or RGECO1.42) However, recently, this concept has been challenged by the data that primary cilia do not respond to mechanical stimuli.45) In the reported study, the dominant Ca2+ oscillation in the left side of the node was not observed in contrast to the previous work.40) Therefore, although Ca2+ increases in the KV at the 4-6 ss and is required for left-right asymmetry, it is still unclear how an increased Ca2+ in the KV could result in left-right asymmetry, which determines the position of organs including the heart.
The node of mice corresponding to the KV of zebrafish determines the left-right asymmetry by nodal flow similar to the flow of the KV. Thus, the defect in nodal cilia results in the misplacement of organs in the body. Mouse nodal cilia lack the central pair of microtubules although they have nine pairs of microtubules with dynein arms. Therefore, cilia are grouped into three classes; normal motile (9+2) cilia, nodal cilia (9+0), and nonmotile (primary or sensory) cilia (9+0).46) Mutations in molecules that constitute cilia lead to ciliopathies.47) Therefore, several organ abnormalities are found in the patients with cilia-related genes. One of the best studied among the ciliopathies is primary ciliary dyskinesia (PCD). About 50% of patients with PCD exhibit abnormal organ positioning; heterotaxy and situs inversus, while the rest show situs solitus.48) The situs inversus is ascribed to the abnormal function of nodal cilia. Kartagener syndrome belongs to PCD and is caused by mutation in DNAI1 and DNAH5.49) Mutations of other ciliopathy-related genes cause CHD, although the organ positioning seems to be situs solitus. Consistently, forward genetic screening of mice reveals that most of the CHD are associated with mutations of cilia-related genes.47)
Atrial and ventricular CPCs that might not be fully differentiated migrate towards the midline to form a cardiac cone.50) As described above, CPCs are specified and differentiated during the blastula stage and the gastrula stage. Even after receiving the signals from left-right patterning molecules, these myocardial precursor cells might undergo fully differentiation process to become mature working cardiomyocytes. Myocardial precursor cells that are located in the bilateral lateral plate mesoderm move between the endoderm and the yolk syncytial layer (YSL) and migrate towards the midline (Fig. 4). Therefore, the defect in the endoderm and the signaling from YSL affects the migration of CPCs. A mutant of endoderm-specific transcription factor, sox32 (casanova) exhibits endodermal defects. Therefore, the defect in endoderm results in the inhibition of CPC migration towards midline, which ultimately leads to cardia bifida (two hearts).33)51)
A mutation in fibronectin (natter) causes cardia bifida, suggesting the importance of extracellular matrix for CPC migration.52) Notably, two mutations in molecules that function in sphingosine-1-phosphate (S1P)-mediated signaling cause cardia bifida: one is mil in which S1P receptor 2 (S1P2) gene is mutated;53) and the other is spsn2 in which S1P transporter gene is mutated.54)55)
It is of note that cardiomyocytes of zebrafish originate from first heart field (FHF) and second heart field (SHF) similar to mammalians.56) The draculin (drl) promoter-activated lateral plate mesodermal cells constitute FHF-derived cardiomyocytes when cardiac tube forms. On the other hand, the latent Tgfβ-binding protein 3 (ltbp3) promoter-activated cells become SHF-derived cardiomyocytes.57) The SHF-derived cardiomyocytes are positive for a homeobox transcription factor, Islet-1, in mammals as well as zebrafish. Although Ltbp3-postive cardiomyocytes are present in the arterial pole of the heart, other ltbp3-positive cells become both smooth muscle cells and endothelial cells. Because the venous pole cells are thought to originate from the SHF as evidenced by the fact that they are positive for Islet-1, it should be clarified how Ltbp3 contributes to the differentiation of the SHF precursors to SHF-derived cardiomyocytes as well as how the Ltbp3-negative cells contribute to the SHF formation.
We have investigated as how S1P signaling regulates the migration of CPCs. Since we identified Spns2 as a S1P transporter functioning in the YSL, we had tried to investigate whether S1P-S1P2 signal is activated and found that the S1P released from the YSL activates S1P2 expressed in the endoderm.58) Spns2 mutant embryos showed the defect in the anterior endoderm where CPCs migrate towards the midline. Consistently, when S1P2 expression was reduced by antisense morpholino oligonucleotide, the morphant exhibited the endodermal defects similarly to the Spns2 mutant. We further demonstrated that the activation of S1P2-dependent signal induces nuclear translocation of Yap, a target of Hippo signaling. Therefore, we concluded that S1P released from the YSL activates S1P2 expressed in the endoderm, thereby inducing nuclear translocation of Yap to maintain the endodermal sheet that is required for CPC migration (Summarized in Fig. 5). Our results highlight the importance of the endoderm in a non-cell autonomous manner when the CPCs migrate towards the midline.
We have reviewed S1P-dependent signaling in the cardiogenesis of zebrafish. S1P-S1P receptor signaling remains elusive in cardiogenesis of mammals, although it has been extensively investigated in vascular development.59)60) Because cardiomyocytes, especially, trabecular cardiomyocytes express S1P1 receptors, cardiomyocyte-specific deletion of other S1P receptor genes will provide us with the information about S1P-dependent signaling in cardiogenesis.61)
As we described the importance of the endoderm when the KV forms and during both blastula and gastrula stages for the allocation and the specification of CPCs, the cardiac development is affected by the other tissues and organs. The signaling from the heart to other tissues might function vice-versa to precisely coordinate the whole body planning.
Figures and Tables
Fig. 1
Mechanisms underlying left-right axis determination. DFCs derived from the endoderm form the KV. The rotation of cilia promotes unidirectional flow in the KV, thereby inducing left-sided specific nodal-related genes. These gene expressions determine the allocation of LPM-derived organs as well as that of the endoderm-derived organs. DFCs: dorsal forerunner cells, KV: Kupffer's vesicle, LPM: lateral plate mesoderm, R: right, L: left.
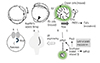
Fig. 2
DFC migration. DFCs can be marked by sox17 promoter activity. The sequential images of Tg (sox17:gfp) from the bud stage (0 h). The elapsed time is indicated at the left top of each image. Arrow heads indicate the DFCs. Arrow indicate the KV. DFC: dorsal forerunner cell, KV: Kupffer's vesicle.
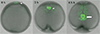
Fig. 3
The Kupffer's vesicle. A Tg (sox1:gfp) embryo at 6 somite stage was immunostained with anti-acetylated tubulin (red). Note that GFP-positive cells are KV-constituting cells with cilia positive for acetylated tubulin. GFP: green fluorescent protein, KV: Kupffer's vesicle.
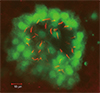
Fig. 4
CPC migration towards the midline. The fate-determined CPCs located in bilateral LPM move towards the midline to form cardiac cone around 22 hfp (upper panel). They migrate in the region sandwiched by the endoderm and the YSL. hpf: hours post fertilization, CPC: cardiac precursor cell, LPM: lateral plate mesoderm, YSL: yolk syncytial layer.
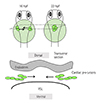
Fig. 5
S1P-regulated proper endoderm formation that is required for CPC migration. S1P released from the YSL activate S1P2 receptor expressed in the endodermal cells. The activated S1P2-mediated signal, then, promotes the translocation of Yap1 into the nucleus. Yap1-promoted Ctgfa. Ctgfa maintains the endoderm that becomes the foothold for CPC migration. S1P: sphingosine 1-phosphate, CPC: cardiac precursor cell, YSL: yolk syncytial layer, Ctgfa: connective tissue growth factor gene A.
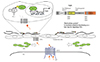
Acknowledgments
We thank Profs. Myeong-Chan Cho and Byung-Su Yoo for their insightful comments on the developmental biology. We also thank Dr. Hyun-Young Park at the Korean NIH for pointing out the importance of Health, Labor, and Welfare Administration in child health in Japan and Korea.
References
1. Regan L, Rai R. Epidemiology and the medical causes of miscarriage. Baillieres Best Pract Res Clin Obstet Gynaecol. 2000; 14:839–854.
2. Wilcox AJ, Weinberg CR, O'Connor JF, et al. Incidence of early loss of pregnancy. N Engl J Med. 1988; 319:189–194.
3. Hoffman JI, Kaplan S. The incidence of congenital heart disease. J Am Coll Cardiol. 2002; 39:1890–1900.
4. Moller JH, Taubert KA, Allen HD, Clark EB, Lauer RM. Cardiovascular health and disease in children: current status. A Special Writing Group from the Task Force on Children and Youth, American Heart Association. Circulation. 1994; 89:923–930.
5. Tong YF. Mutations of NKX2.5 and GATA4 genes in the development of congenital heart disease. Gene. 2016; 588:86–94.
6. McCulley DJ, Black BL. Transcription factor pathways and congenital heart disease. Curr Top Dev Biol. 2012; 100:253–277.
7. Ramsdell AF. Left-right asymmetry and congenital cardiac defects: getting to the heart of the matter in vertebrate left-right axis determination. Dev Biol. 2005; 288:1–20.
8. Patton EE, Zon LI. The art and design of genetic screens: zebrafish. Nat Rev Genet. 2001; 2:956–966.
9. Santoriello C, Zon LI. Hooked! Modeling human disease in zebrafish. J Clin Invest. 2012; 122:2337–2343.
10. Auer TO, Del BF. CRISPR/Cas9 and TALEN-mediated knock-in approaches in zebrafish. Methods. 2014; 69:142–150.
11. Driever W, Fishman MC. The zebrafish: heritable disorders in transparent embryos. J Clin Invest. 1996; 97:1788–1794.
12. Chen JN, Fishman MC. Genetics of heart development. Trends Genet. 2000; 16:383–388.
13. Yelon D. Cardiac patterning and morphogenesis in zebrafish. Dev Dyn. 2001; 222:552–563.
14. Rottbauer W, Wessels G, Dahme T, et al. Cardiac myosin light chain-2: a novel essential component of thick-myofilament assembly and contractility of the heart. Circ Res. 2006; 99:323–331.
15. Guner-Ataman B, Paffett-Lugassy N, Adams MS, et al. Zebrafish second heart field development relies on progenitor specification in anterior lateral plate mesoderm and nkx2.5 function. Development. 2013; 140:1353–1363.
16. Lazic S, Scott IC. Mef2cb regulates late myocardial cell addition from a second heart field-like population of progenitors in zebrafish. Dev Biol. 2011; 354:123–133.
17. Schier AF, Talbot WS. Molecular genetics of axis formation in zebrafish. Annu Rev Genet. 2005; 39:561–613.
18. D'Amico LA, Cooper MS. Spatially distinct domains of cell behavior in the zebrafish organizer region. Biochem Cell Biol. 1997; 75:563–577.
19. Matsui T, Ishikawa H, Bessho Y. Cell collectivity regulation within migrating cell cluster during Kupffer's vesicle formation in zebrafish. Front Cell Dev Biol. 2015; 3:27.
20. Okabe N, Xu B, Burdine RD. Fluid dynamics in zebrafish Kupffer's vesicle. Dev Dyn. 2008; 237:3602–3612.
21. Kramer-Zucker AG, Olale F, Haycraft CJ, Yoder BK, Schier AF, Drummond IA. Cilia-driven fluid flow in the zebrafish pronephros, brain and Kupffer's vesicle is required for normal organogenesis. Development. 2005; 132:1907–1921.
22. Long S, Ahmad N, Rebagliati M. The zebrafish nodal-related gene southpaw is required for visceral and diencephalic left-right asymmetry. Development. 2003; 130:2303–2316.
23. Keegan BR, Meyer D, Yelon D. Organization of cardiac chamber progenitors in the zebrafish blastula. Development. 2004; 131:3081–3091.
24. Keegan BR, Feldman JL, Begemann G, Ingham PW, Yelon D. Retinoic acid signaling restricts the cardiac progenitor pool. Science. 2005; 307:247–249.
25. Ueno S, Weidinger G, Osugi T, et al. Biphasic role for Wnt/beta-catenin signaling in cardiac specification in zebrafish and embryonic stem cells. Proc Natl Acad Sci USA. 2007; 104:9685–9690.
26. Marques SR, Lee Y, Poss KD, Yelon D. Reiterative roles for FGF signaling in the establishment of size and proportion of the zebrafish heart. Dev Biol. 2008; 321:397–406.
27. Marques SR, Yelon D. Differential requirement for BMP signaling in atrial and ventricular lineages establishes cardiac chamber proportionality. Dev Biol. 2009; 328:472–482.
28. Reiter JF, Verkade H, Stainier DY. Bmp2b and Oep promote early myocardial differentiation through their regulation of gata5. Dev Biol. 2001; 234:330–338.
29. Zeng XX, Wilm TP, Sepich DS, Solnica-Krezel L. Apelin and its receptor control heart field formation during zebrafish gastrulation. Dev Cell. 2007; 12:391–402.
30. Reiter JF, Alexander J, Rodaway A, et al. Gata5 is required for the development of the heart and endoderm in zebrafish. Genes Dev. 1999; 13:2983–2995.
31. Begemann G, Schilling TF, Rauch GJ, Geisler R, Ingham PW. The zebrafish neckless mutation reveals a requirement for raldh2 in mesodermal signals that pattern the hindbrain. Development. 2001; 128:3081–3094.
32. Essner JJ, Amack JD, Nyholm MK, Harris EB, Yost HJ. Kupffer's vesicle is a ciliated organ of asymmetry in the zebrafish embryo that initiates left-right development of the brain, heart and gut. Development. 2005; 132:1247–1260.
33. Kikuchi Y, Agathon A, Alexander J, et al. Casanova encodes a novel Sox-related protein necessary and sufficient for early endoderm formation in zebrafish. Genes Dev. 2001; 15:1493–1505.
34. Amack JD, Yost HJ. The T box transcription factor no tail in ciliated cells controls zebrafish left-right asymmetry. Curr Biol. 2004; 14:685–690.
35. Mizoguchi T, Verkade H, Heath JK, Kuroiwa A, Kikuchi Y. Sdf1/Cxcr4 signaling controls the dorsal migration of endodermal cells during zebrafish gastrulation. Development. 2008; 135:2521–2529.
36. Matsui T, Thitamadee S, Murata T, et al. Canopy1, a positive feedback regulator of FGF signaling, controls progenitor cell clustering during Kupffer's vesicle organogenesis. Proc Natl Acad Sci USA. 2011; 108:9881–9886.
37. Neugebauer JM, Amack JD, Peterson AG, Bisgrove BW, Yost HJ. FGF signalling during embryo development regulates cilia length in diverse epithelia. Nature. 2009; 458:651–654.
38. Tanaka Y, Okada Y, Hirokawa N. FGF-induced vesicular release of Sonic hedgehog and retinoic acid in leftward nodal flow is critical for left-right determination. Nature. 2005; 435:172–177.
39. Pennekamp P, Karcher C, Fischer A, et al. The ion channel polycystin-2 is required for left-right axis determination in mice. Curr Biol. 2002; 12:938–943.
40. Takao D, Nemoto T, Abe T, et al. Asymmetric distribution of dynamic calcium signals in the node of mouse embryo during left-right axis formation. Dev Biol. 2013; 376:23–30.
41. Sarmah B, Latimer AJ, Appel B, Wente SR. Inositol polyphosphates regulate zebrafish left-right asymmetry. Dev Cell. 2005; 9:133–145.
42. Yuan S, Zhao L, Brueckner M, Sun Z. Intraciliary calcium oscillations initiate vertebrate left-right asymmetry. Curr Biol. 2015; 25:556–567.
43. Roxo-Rosa M, Jacinto R, Sampaio P, Lopes SS. The zebrafish Kupffer's vesicle as a model system for the molecular mechanisms by which the lack of Polycystin-2 leads to stimulation of CFTR. Biol Open. 2015; 4:1356–1366.
44. Lahvic JL, Ji Y, Marin P, et al. Small heat shock proteins are necessary for heart migration and laterality determination in zebrafish. Dev Biol. 2013; 384:166–180.
45. Delling M, Indzhykulian AA, Liu X, et al. Primary cilia are not calcium-responsive mechanosensors. Nature. 2016; 531:656–660.
46. Knowles MR, Daniels LA, Davis SD, Zariwala MA, Leigh MW. Primary ciliary dyskinesia. Recent advances in diagnostics, genetics, and characterization of clinical disease. Am J Respir Crit Care Med. 2013; 188:913–922.
47. Rao DR, Gabriel GC, Li Y, et al. Role of cilia in structural birth defects: insights from ciliopathy mutant mouse models. Birth Defects Res C Embryo Today. 2014; 102:115–125.
48. Kennedy MP, Omran H, Leigh MW, et al. Congenital heart disease and other heterotaxic defects in a large cohort of patients with primary ciliary dyskinesia. Circulation. 2007; 115:2814–2821.
49. McGrath J, Brueckner M. Cilia are at the heart of vertebrate left-right asymmetry. Curr Opin Genet Dev. 2003; 13:385–392.
50. Stainier DY, Lee RK, Fishman MC. Cardiovascular development in the zebrafish I Myocardial fate map and heart tube formation. Development. 1993; 119:31–40.
51. Dickmeis T, Mourrain P, Saint-Etienne L, et al. A crucial component of the endoderm formation pathway, CASANOVA, is encoded by a novel sox-related gene. Genes Dev. 2001; 15:1487–1492.
52. Sakaguchi T, Kikuchi Y, Kuroiwa A, Takeda H, Stainier DY. The yolk syncytial layer regulates myocardial migration by influencing extracellular matrix assembly in zebrafish. Development. 2006; 133:4063–4072.
53. Kupperman E, An S, Osborne N, Waldron S, Stainier DY. A sphingosine-1-phosphate receptor regulates cell migration during vertebrate heart development. Nature. 2000; 406:192–195.
54. Kawahara A, Nishi T, Hisano Y, Fukui H, Yamaguchi A, Mochizuki N. The sphingolipid transporter spns2 functions in migration of zebrafish myocardial precursors. Science. 2009; 323:524–527.
55. Osborne N, Brand-Arzamendi K, Ober EA, et al. The spinster homolog, two of hearts, is required for sphingosine 1-phosphate signaling in zebrafish. Curr Biol. 2008; 18:1882–1888.
56. Liu J, Stainier DY. Zebrafish in the study of early cardiac development. Circ Res. 2012; 110:870–874.
57. Zhou Y, Cashman TJ, Nevis KR, et al. Latent TGF-beta binding protein 3 identifies a second heart field in zebrafish. Nature. 2011; 474:645–648.
58. Fukui H, Terai K, Nakajima H, Chiba A, Fukuhara S, Mochizuki N. S1P-Yap1 signaling regulates endoderm formation required for cardiac precursor cell migration in zebrafish. Dev Cell. 2014; 31:128–136.
59. Mendelson K, Evans T, Hla T. Sphingosine 1-phosphate signalling. Development. 2014; 141:5–9.
60. Xiang SY, Dusaban SS, Brown JH. Lysophospholipid receptor activation of RhoA and lipid signaling pathways. Biochim Biophys Acta. 2013; 1831:213–222.
61. Clay H, Wilsbacher LD, Wilson SJ, et al. Sphingosine 1-phosphate receptor-1 in cardiomyocytes is required for normal cardiac development. Dev Biol. 2016; 418:157–165.