Abstract
Background and Objectives
Differentiation and de-differentiation of vascular smooth muscle cells (VSMCs) are important events in atherosclerosis and restenosis after angioplasty. MicroRNAs are considered a key regulator in cellular processes such as differentiation, proliferation, and apoptosis. Here, we report the role of new miR-18a-5p microRNA and its downstream target genes in VSMCs and in a carotid balloon injury model.
Materials and Methods
Expression of miR-18a-5p and its candidate genes was examined in VSMCs and in a carotid artery injury model by quantitative reverse-transcription polymerase chain reaction (qRT-PCR) and microRNA microarray analysis. VSMC differentiation marker genes including smooth muscle (SM) α-actin and SM22α were determined by Western blot, qRT-PCR, and a SM22α promoter study. Gene overexpression or knockdown was performed in VSMCs.
Results
miR-18a-5p was upregulated in the rat carotid artery at the early time after balloon injury. Transfection of the miR-18a-5p mimic promoted the VSMC differentiation markers SM α-actin and SM22α. In addition, miR-18a-5p expression was induced in differentiated VSMCs, whereas it decreased in de-differentiated VSMCs. We identified syndecan4 as a downstream target of miR-18-5p in VSMCs. Overexpression of syndecan4 decreased Smad2 expression, whereas knockdown of syndecan4 increased Smad2 expression in VSMCs. Finally, we showed that Smad2 induced the expression of VSMC differentiation marker genes in VSMCs.
Vascular smooth muscle cells (VSMCs) are major cells in the neointimal region and play a role in vascular diseases.1) Unlikely other cells, VSMCs can switch phenotypes between contractile (differentiation) and proliferative (de-differentiation) state in response to various pathological stimuli. This vascular phenotype switching contributes to the development of cardiovascular diseases such as atherosclerosis, restenosis, and hypertension.2) Therefore, regulating the VSMC phenotype may have potential as a therapeutic target for treating pathological vascular diseases.
The most significant breakthrough in modulating gene expression is the discovery of microRNAs. MicroRNAs (miRs) are small, non-coding RNAs that negatively regulate gene expression by degradation or translational inhibition of microRNA target genes.3)4) MicroRNAs are currently considered key regulators or possible biomarkers of various pathological diseases, ranging from various cancers to cardiovascular diseases. They are expressed in a cell- or tissue-specific manner and play important roles in many cellular processes such as cell differentiation, proliferation/growth, apoptosis, and migration. Recent studies have shown that microRNAs are phenotypic regulators of VSMCs. For example, miR-663, miR-221, miR-143, miR-145, miR-133, and miR-26 regulate VSMC differentiation and phenotypic changes.5)6)7)8)9)10) Our group has studied the roles of microRNAs in VSMCs as well as in a rat carotid injury model. We reported that miR-132 induces VSMC differentiation and that miR-132 delivery reduces neointimal hyperplasia.11) Several studies have revealed that miR-18a-5p is involved in development,12) proliferation,13) and differentiation.14) However, the role of miR-18a-5p in VSMC proliferation and differentiation is currently unknown.
In the present study, we identified new microRNAs that regulate VSMC differentiation in a rat carotid artery balloon injury model. We also demonstrated that miR-18a-5p promotes VSMC differentiation by downregulating syndecan4.
Human recombinant platelet derived growth factor-BB (PDGF-BB) was purchased from Sigma (St. Louis, MO, USA). MiRNA-18a-5p and the miRNA control were purchased from Bioneer Co. (Daejeon, Korea).
Gapdh (sc-32233), syndecan4 (sc-15350), smooth muscle (SM) 22α (sc-373928), and SM α-actin (sc-130617) were purchased from Santa Cruz Biotechnology (Santa Cruz, CA, USA). Smad2 (#3103) was purchased from Cell Signaling Technology (Danvers, MA, USA).
Rat carotid injury experiments were performed as described previously. Briefly, 8-week-old male Sprague-Dawley rats (n=8, Damul, Daejeon, Korea) were used, and all experimental procedures were approved by the Chonnam National University Medical School Research Institutional Animal Care and Use Committee. Rats were anesthetized for balloon injury, and the right common carotid artery was exposed by a midline cervical incision. A 2 Fr Fogarty balloon catheter was placed at the puncture site of the external carotid artery and retracted three times under mild balloon inflation pressure to denude the endothelium. The left carotid artery was used as the sham control. The arteries were harvested at 1, 7, and 14 days after injury. microRNA expression profiles were analyzed with an Agilent microRNA microarray (Agilent Technologies, Palo Alto, CA, USA).
Vascular smooth muscle cells were isolated from the aortic media of male rats by enzymatic dissociation as described previously.15) VSMCs were used at passages 5-11. A10 cells were obtained from the American Type Culture Collection (Manassas, VA, USA). The cells were grown in Dulbecco's modified Eagle's medium (DMEM) with 10% fetal bovine serum (FBS).
Vascular smooth muscle cell differentiation was induced by serum deprivation. Briefly, cells were seeded into 6-well plates at 50% confluence and maintained in 0.5% DMEM media containing 0.5% FBS for 4 days. Cells were serum-starved for 24 hours and treated with PDGF-BB (25 ng/mL) for 3 days to de-differentiate.
Double-stranded RNAs were designed to overexpress the endogenous mature miR-18a-5p. Primary VSMCs were transfected with miR-18a-5p mimic or negative control miRNA (75 nM, Bioneer) using the RNAiMax reagent (Invitrogen, Carlsbad, CA, USA) according to the manufacturer's protocol.
pCMV-SPORT6-Smad2 was obtained from KUGI (Seoul, Korea). The pGL3-441 SM22α promoter-luciferase construct was kindly provided by Prof. Michael S. Parmacek (University of Pennsylvania, Philadelphia, PA, USA). The pcDNA-syndecan4 full wild construct was kindly provided by Prof. Eok-Soo Oh (Ewha Womans University, Seoul, Korea). All plasmids were confirmed by sequencing.
Syndecan4 knockdown was performed using its siRNA (si-syndecan4, 50 nM, cat no. sc-270178, Santa Cruz Biotechnology, Santa Cruz, CA, USA). An siRNA targeting rat syndecan4 was transfected into A10 cells using the RNAiMax reagent. Nontargeting siRNA (Bioneer) was used as a negative control.
Cells (A10 and 293T) were seeded in 24-well plates 18 hours before transfection. The pGL3-441 SM22α promoter and pCMV-β-galactosidase were transfected with Lipofectamine Plus reagent (Invitrogen, Carlsbad, CA, USA) or PolyFect reagent (Qiagen, Valencia, CA, USA), according to the manufacturer's instructions. The cells were harvested 48 hours after transfection, and luciferase activity was measured and normalized against β-galactosidase activity as an internal control.
Cells were harvested with RIPA lysis buffer as described previously.15) Cell extracts were subjected to sodium dodecyl sulfate-polyacrylamide gel electrophoresis and transferred to a PVDF membrane. Western blotting was performed using anti-SM α-actin, anti-SM22α, anti-syndecan4, anti-Smad2, and anti-GAPDH. The blots were developed using Immobilon Western Detection Reagents (Millipore, Billerica, MA, USA).
Total RNAs were extracted with a Trizol kit according to the manufacturer's instructions.
Real time polymerase chain reaction (RT-PCR) was performed as described previously.16) Polymerase chain reaction (PCR) was performed using the following oligonucleotide primers: for SM α-actin, sense, 5'-AGTCGCCATCAGGAACCTCGAGAA-3', and antisense, 5'-GC CAGATCTTTTCCATGTCGTCCC-3'; for SM22α, sense, 5'-CCCACAAAC GACCAAGCCTTTTCT-3', and antisense, 5'-CCTGTTCCATCTGCT GAAGACCA-3'; for calponin, sense, 5'-ACAACACCCAAAGGAAGCAC-3', and antisense, 5'-TCACTGCAAAACCAAACTGC-3'; for Smad2, sense, 5'-CCAGGTCTCTTGATGGTCGT-3', and antisense, 5'-ACTGGTGTCTC CACCCTCTG-3'; for syndecan4, sense, 5'-GATAACCACATCCCC GAGAA-3', and antisense, 5'-CACAATCAGAGCTGCCAAGA-3'; for glyceraldehyde-3-phosphate dehydrogenase, sense, 5'-AACCCAT CACCATCTTCCAGGAGC-3', and antisense, 5'-ATGGACTGTGGTCAT GAGCCCTTC-3';
RNAs from rat carotid arteries and VSMCs were isolated with TRIzol (Invitrogen, Carlsbad, CA, USA) to detect mature miR-18a-5p levels. qRT-PCR for miR-18a-5p was performed using cDNA generated from 10 ng of total RNA using the TaqMan MicroRNA Reverse Transcription kit (part no. 4366596, Applied Biosystems, Foster City, CA, USA). 18S was used as an internal control. Quantification of the mRNA amounts was done with SYBR Green PCR kit (Applied Biosystems, Foster, CA, USA).
We used the miR Base Target database to find putative miR-18a-5p targets (www.mirbase.org). Candidate target genes were determined by qRT-PCR.
To explore the role of miR-18a-5p in the rat carotid injury model and VSMCs, we first investigated miR-18a-5p expression in carotid arteries at 1, 7, and 14 days after injury. Using microRNA qRT-PCR analysis, we observed induction of miR-18a-5p 1 day after carotid injury compared with the sham control (Fig. 1A). Next, we evaluated whether miR-18a-5p affected proliferation or differentiation of VSMCs. MiR-18a-5p mimic did not affect proliferation of VSMCs (data not shown). As shown in Fig. 1B, C, and D, the miR-18a-5p mimic increased SMα-actin and SM22α protein amounts. We further determined other VSMC differentiation markers using qRT-PCR. SM α-actin, SM 22α, and calponin mRNA levels were induced by transfection of the miR-18a-5p mimic into VSMCs (Fig. 1E, F, and G).
Unexpectedly, the qRT-PCR analysis revealed enhanced SM22α and SMα-actin mRNA levels in injured arteries compared with those in sham-operated arteries (Fig. 2A and B). We determined the expression of miR-18a-5p and differentiation markers during differentiation and de-differentiation of VSMCs to verify whether miR-18a-5p was involved in the VSMC phenotype. We found that SM22α protein expression was induced in VSMC differentiation medium (Fig. 2C). SM α-actin and SM22α mRNA levels increased in differentiated VSMCs (Fig. 2D and E). As shown in Fig. 2F, miR-18a-5p expression increased after exposure to differentiation medium. De-differentiated VSMCs induced by treatment with PDGF-BB showed decreased SM22α and SM α-actin mRNA levels. In addition, SM α-actin protein expression decreased in PDGF-BB-treated VSMCs (Fig. 2I). Interestingly, we found that miR-18a-5p expression was downregulated in PDGF-BB-stimulated VSMCs (Fig. 2J).
We used bioinformatics tools to identify candidate miR-18a-5p target genes. Considering that miR-18a-5p is involved in VSMC differentiation, we hypothesized that downstream miR-18a-5p target genes might be associated with differentiation. Therefore, we selected eight genes (NFAT5, Hiflα, Hsf2, Tmx4, CTGF, KLF6, syndecan4, and Smad2). These candidate genes have been reported to relate to differentiation.
We first evaluated whether miR-18a-5p expression was induced by transfection of the miR-18a-5p mimic into VSMCs (Fig. 3A). Next, we tested whether miR-18a-5p downregulated expression of the candidate target genes. Although Smad2 contains the miR-18a-5p binding site in the Smad2 3' untranslated region, Smad2 protein levels remained unchanged in miR-18a-5p-transfected VSMCs (Fig. 3B). This result indicates that Smad2 is not a direct target of miR-18a-5p. Of the genes examined, syndecan4 mRNA levels decreased significantly in both VSMCs and A10 cells transfected with miR-18a-5p (Fig. 3C and D). In addition, syndecan4 protein expression was clearly reduced in VSMCs transfected with miR-18a-5p (Fig. 3E). Quantification of syndecan4 protein levels is shown in Fig. 3F. To further identify whether syndecan4 was a target of miR-18a-5p in balloon-injured rat carotid artery, we examined syndecan4 mRNA expression levels by qRT-PCR. As shown in Fig. 3G, syndecan4 mRNA was highly expressed 7 days after balloon injury. In addition, we found that Smad2 expression was enhanced 7 days after carotid injury (Fig. 3H).
To identify whether syndecan4 is involved in VSMC differentiation, we examined differentiation marker genes in VSMCs. We confirmed that the amounts of syndecan4 mRNA and protein largely increased by transfection with the syndecan4 expression vector in A10 cells (Fig. 4A and E). However, the SM differentiation markers SM22α and SM α-actin remained unchanged by overexpressing syndecan4 in A10 cells (Fig. 4B and C). Unexpectedly, we found that Smad2 protein expression decreased in syndecan4-overexpressing cells (Fig. 4D and F). To further determine regulation of Smad2 by syndecan4, the cells were transfected with VSMCs with syndecan4 siRNA. Endogenous syndecan4 decreased by syndecan4 siRNA treatment (Fig. 4G and H). Despite the significant reduction in syndecan4, syndecan4 siRNA did not affect the smooth muscle differentiation markers (Fig. 4G). However, Smad2 expression increased slightly by syndecan4 siRNA (Fig. 4I).
Although the miRBase prediction database showed several miR-18a-5p target genes, Smad2 did not decrease following transfection with the miR-18a-5p mimic. Because Smad2 was downregulated by syndecan4 in VSMCs, we sought to determine whether Smad2 was involved in differentiation. We transiently transfected pCMV-SPORT6-Smad2 or empty vector into A10 cells and examined Smad2 protein expression. When cells were transfected with Smad2, SM α-actin and SM22α protein expression increased (Fig. 5A-D). SM 22α is a differentiation marker. Cells were transfected with the -441 SM22α minimal promoter construct in the presence or absence of Smad2. As shown in Fig. 5E and F, exogenous Smad2 significantly activated the SM22α promoter in both A10 and 293T cells.
We showed that miR-18a-5p was upregulated at the early phase after rat carotid balloon injury and promoted VSMC differentiation. The main observation of our study is that miR-18a-5p promoted VSMC differentiation by inhibiting syndecan4 expression. Although syndecan4 did not directly affect VSMC differentiation, miR-18a-5p-repressed syndecan4 induced Smad2, which activated VSMC differentiation (Fig. 5G). The detailed molecular mechanism needs to be clarified in a future study.
We demonstrated that miR-18a-5p promoted the expression of VSMC differentiation marker genes (i.e., SM α-actin and SM22α). In addition, we found that miR-18a-5p expression was upregulated at the early time point after balloon injury. We further demonstrated that miR-18a-5p expression increased during VSMC differentiation, whereas it decreased in VSMC de-differentiation medium. Therefore, we suggest that miR-18a-5p is a novel modulator of the VSMC phenotypic switch.
Considering the effect of VSMC differentiation by miR-18a-5p, we hypothesized that miR-18a-5p must downregulate anti-differentiation genes. Among several genes, we found that syndecan4 mRNA and protein expression were markedly downregulated by miR-18a-5p in VSMCs. However, the downregulation of syndecan4 by overexpression of miR-18a-5p in VSMCs was not completely mimicked in the rat carotid injury model. In our animal experiment, the syndecan4 transcript level was dramatically enhanced in injured arteries 7 days after carotid injury. The reason is attributed to a decrease in miR-18a-5p expression over time after carotid injury.
Syndecan4 is a family of heparan sulfate proteoglycan. Studies have demonstrated that syndecan4 is involved in cardiovascular diseases, such as cardiac hypertrophy and myocardial infarction as well as arterial restenosis after angioplasty.17)18) Herum et al.19) reported that syndecan4 is involved in differentiation from cardiac fibroblasts to myofibroblasts in response to mechanical stress such as pressure overload. However, our results show that transfection of A10 cells with syndecan4 did not affect VSMC differentiation markers. Our results suggest that syndecan4 does not act as an inducer of VSMC differentiation. Syndecan4 could regulate expression of differentiation-associated genes as a possible mechanism of miR-18a-5p-induced VSMC differentiation.
Although syndecan4 is a candidate target of miR-18a-5p, it does not show VSMC differentiation characteristics. Therefore, we investigated whether Smad2 is a downstream gene of syndecan4 in VSMCs. We found that syndecan4 regulates Smad2 expression in VSMCs. Indeed, overexpression of syndecan4 reduced Smad2 protein expression, whereas knockdown of syndecan4 increased expression of Smad2. Xie et al. showed that Smad2 acts as a critical regulator of VSMC differentiation in neural crest cells.20) We found that Smad2 induced protein expression and promoter activity of SM22α in VSMCs.
In conclusion, we identified miR-18a-5p as a novel regulator of VSMC differentiation. Our findings suggest that miR-18a-5p/Smad2 may be a potential therapeutic for treating pathological vascular diseases such as atherosclerosis and in-stent restenosis.
Figures and Tables
Fig. 1
Vascular smooth muscle cell (VSMC) differentiation marker genes increased in VSMCs transfected with miR-18a-5p. A: miR-18a-expression 5p was induced in injured rat carotid arteries compared with that in contralateral uninjured tissues. B: VSMCs were transfected with control or miR-18a-5p mimic. The expression of smooth muscle (SM) α-actin and SM22α was determined by Western blot analysis 3 days after transfection. C and D: quantification of SM α-actin and SM22α from three different sets of Western blots. E, F, and G: expression of SM α-actin, SM22α, and calponin normalized to glyceraldehyde-3-phosphate dehydrogenase was examined by quantitative reverse transcription-polymerase chain reaction. Values are the means±standard errors (n=4-6 per group). p<0.05, p<0.01, and p<0.001 compared with sham or control group.
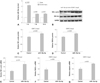
Fig. 2
miR-18a-5p expression during differentiation and de-differentiation. A and B: the mRNA levels of smooth muscle (SM)22α and SM α-actin were measured in a rat carotid injury model by quantitative reverse transcription-polymerase chain reaction (qRT-PCR). C: vascular smooth muscle cell (VSMC) differentiation (4 days) was induced by serum deprivation. The SM22α protein was highly expressed in cultured VSMCs in differentiation medium {DM, DMEM with 0.5% fetal bovine serum (FBS)}. GM, VSMCs were incubated with 10% FBS in DMEM. D and E: SM α-actin and SM22α mRNA level were measured by qRT-PCR. F: miR-18a-5p expression was evaluated in VSMCs cultured DM for 4 days. G and H: for de-differentiation induction, VSMCs were treated with platelet derived growth factor (PDGF)-BB for 3 days. SM α-actin and SM22α mRNA level were determined by qRT-PCR. I: SM α-actin protein was determined by Western blot analysis. J: miR-18a-5p expression was measured in response to PDGF-BB in VSMCs. Values are means±standard errors (n=4-6 per group). p<0.05, p<0.01, and p<0.001 compared with sham, vehicle, and GM group, respectively, GM: growth medium (DMEM with 10% FBS).
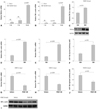
Fig. 3
Syndecan4 expression is down-regulated in vascular smooth muscle cells (VSMCs) transfected with miR-18a-5p. A: VSMCs or A10 cells were transfected with control miR or miR-18a-5p mimic. Three days later, the cells were subjected to quantitative reverse transcription-polymerase chain reaction. B, C, and D: Smad2 and syndecan4 mRNA was evaluated in cells transfected with the miR-18a-5p mimic. E: Smad2 and syndecan4 protein expression was determined by Western blot. F: quantification of syndecan4 protein levels. Syndecan4 (G) and Smad2 (H) mRNA levels were measured in sham and balloon-injured arteries at 1, 4, and 7 days after carotid injury.
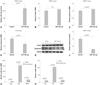
Fig. 4
Syndecan4 decreases Smad2 expression in vascular smooth muscle cells (VSMCs). A, B, and C: syndecan4, smooth muscle (SM)22α, and SM α-actin mRNA levels were determined in A10 cells transfected with pcDNA-syndecan4 (SDC4) or pcDNA empty vector. D: syndecan4, Smad2, SM α-actin, and SM22α protein expression was evaluated by Western blot in A10 cells. E and F: the intensity of syndecan4 and Smad2 protein expression was quantified by densitometry. G: silencing of syndecan4 by specific small interfering RNA (syndecan4 siRNA) reduced syndecan4 expression as determined by Western blot. H and I: Syndecan4 siRNA reduced syndecan4 protein, whereas it increased Smad2 protein expression.
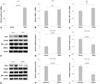
Fig. 5
Smad2 increases vascular smooth muscle cell (VSMC) differentiation marker genes. A: Smad2, smooth muscle (SM) α-actin, and SM22α protein amounts were analyzed in A10 cells transfected with pCMV-SPORT6-Smad2 or pCMV-SPORT6 vector. B, C, and D: Smad2, SM α-actin, and SM22α protein levels were quantified by scanning densitometry. E and F: A10 cells or 293T cells were transfected with -441 SM22α promoter luciferase construct with β-galactosidase. G: schematic diagram of miR-18a-5p induced VSMC differentiation. miR-18a-5p or Smad2 induced VSMC differentiation. Syndecan4 is a downstream target of miR-18a-5p but the mechanism is unknown.
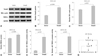
Acknowledgments
This study was supported by a research grant from the Korean Society of Cardiology in 2011.
References
1. Doran AC, Meller N, McNamara CA. Role of smooth muscle cells in the initiation and early progression of atherosclerosis. Arterioscler Thromb Vasc Biol. 2008; 28:812–819.
2. Owens GK, Kumar MS, Wamhoff BR. Molecular regulation of vascular smooth muscle cell differentiation in development and disease. Physiol Rev. 2004; 84:767–801.
3. Ambros V. The functions of animal microRNAs. Nature. 2004; 431:350–355.
4. He L, Hannon GJ. MicroRNAs: small RNAs with a big role in gene regulation. Nat Rev Genet. 2004; 5:522–531.
5. Cordes KR, Sheehy NT, White MP, et al. miR-145 and miR-143 regulate smooth muscle cell fate and plasticity. Nature. 2009; 460:705–710.
6. Cheng Y, Liu X, Yang J, et al. MicroRNA-145, a novel smooth muscle cell phenotypic marker and modulator, controls vascular neointimal lesion formation. Circ Res. 2009; 105:158–166.
7. Li P, Zhu N, Yi B, et al. MicroRNA-663 regulates human vascular smooth muscle cell phenotypic switch and vascular neointimal formation. Circ Res. 2013; 113:1117–1127.
8. Leeper NJ, Raiesdana A, Kojima Y, et al. MicroRNA-26a is a novel regulator of vascular smooth muscle cell function. J Cell Physiol. 2011; 226:1035–1043.
9. Torella D, Iaconetti C, Catalucci D, et al. MicroRNA-133 controls vascular smooth muscle cell phenotypic switch in vitro and vascular remodeling in vivo. Circ Res. 2011; 109:880–893.
10. Davis BN, Hilyard AC, Nguyen PH, Lagna G, Hata A. Induction of microRNA-221 by platelet-derived growth factor signaling is critical for modulation of vascular smooth muscle phenotype. J Biol Chem. 2009; 284:3728–3738.
11. Choe N, Kwon JS, Kim JR, et al. The microRNA miR-132 targets Lrrfip1 to block vascular smooth muscle cell proliferation and neointimal hyperplasia. Atherosclerosis. 2013; 229:348–355.
12. Friedman LM, Dror AA, Mor E, et al. MicroRNAs are essential for development and function of inner ear hair cells in vertebrates. Proc Natl Acad Sci U S A. 2009; 106:7915–7920.
13. Hayashita Y, Osada H, Tatematsu Y, et al. A polycistronic microRNA cluster, miR-17-92, is overexpressed in human lung cancers and enhances cell proliferation. Cancer Res. 2005; 65:9628–9632.
14. Ohgawara T, Kubota S, Kawaki H, et al. Regulation of chondrocytic phenotype by micro RNA 18a: involvement of Ccn2/Ctgf as a major target gene. FEBS Lett. 2009; 583:1006–1010.
15. Kee HJ, Kwon JS, Shin S, Ahn Y, Jeong MH, Kook H. Trichostatin A prevents neointimal hyperplasia via activation of Krüppel like factor 4. Vascul Pharmacol. 2011; 55:127–134.
16. Kwon JS, Joung H, Kim YS, et al. Sulforaphane inhibits restenosis by suppressing inflammation and the proliferation of vascular smooth muscle cells. Atherosclerosis. 2012; 225:41–49.
17. Ikesue M, Matsui Y, Ohta D, et al. Syndecan-4 deficiency limits neointimal formation after vascular injury by regulating vascular smooth muscle cell proliferation and vascular progenitor cell mobilization. Arterioscler Thromb Vasc Biol. 2011; 31:1066–1074.
18. Matsui Y, Ikesue M, Danzaki K, et al. Syndecan-4 prevents cardiac rupture and dysfunction after myocardial infarction. Circ Res. 2011; 108:1328–1339.
19. Herum KM, Lunde IG, Skrbic B, et al. Syndecan-4 signaling via NFAT regulates extracellular matrix production and cardiac myofibroblast differentiation in response to mechanical stress. J Mol Cell Cardiol. 2013; 54:73–81.
20. Xie WB, Li Z, Shi N, et al. Smad2 and myocardin-related transcription factor B cooperatively regulate vascular smooth muscle differentiation from neural crest cells. Circ Res. 2013; 113:e76–e86.