Abstract
Background and Objectives
Increased vascular wall shear stress by elevated plasma viscosity significantly enhances the endothelial nitric oxide synthase (eNOS) activity during an acute isovolemic hemodilution. Also the modulation of plasma viscosity has effects on the cardiac function that were revealed if a left ventricular (LV) pressure-volume (PV) measurement was used. The aim of this study was to assess cardiac function responses to nitric oxide synthase (NOS) inhibitors with the presence of an elevated plasma viscosity but a low hematocrit level. Furthermore, systemic parameters were monitored in a murine model.
Materials and Methods
As test group five anesthetized hamsters were administered with N(G)-nitro-L-arginine methyl ester (L-NAME), NOS inhibitor, whereas five other hamsters were used as control group without L-NAME infusion. The dosage of L-NAME was 10 mg/kg. An isovolemic hemodilution was performed by 40% of estimated blood volume with 6% w/v dextran 2000 kDa, high viscosity plasma expanders (PEs) with viscosity 6.34 cP. LV function was measured and assessed using a 1.4 Fr PV conductance catheter.
Results
The study results demonstrated that NOS inhibition prevented the normal cardiac adaptive response after hemodilution. The endsystolic pressure increased 14% after L-NAME infusion and maintained higher than at the baseline after hemodilution, whereas it gradually decreased in the animals without L-NAME infusion. The admission of L-NAME significantly decreased the maximum rate of ventricular pressure rise (+dP/dtmax), stroke volume and cardiac output after hemodilution if compared to the control group (p<0.05).
Flow-induced shear stress plays a central role on endothelial response and mechano-signal transduction in the cardiovascular system. The mechanical shear force stimulates endothelial protein activities including nitric oxide synthesis (NOS) by endothelial nitric oxide synthase (eNOS).1)2) Nitric oxide (NO) is an important mediator that has various physiological functions such as vascular tone regulation, platelet activation prevention, vascular smooth muscle proliferation inhibition and myocardial contractility regulation.3)4)5) The inhibition of eNOS activity and endothelial dysfunction decreases the inotropic effect of ventricular myocytes and constricts blood vessels in a normal physiological range of hematocrit (Hct).6) Furthermore, the injection of N(G)-nitro-L-arginine methyl ester (L-NAME) or other NOS inhibitors induces high blood pressure and increases microvascular resistance to blood flow.7)8)9)
Vascular wall shear stress (WSS) is modulated by blood flow and blood viscosity. A previous study in the cremaster muscles of hamsters using dextran with different molecular weights as an exchange solution showed that elevated plasma viscosity increased WSS and produced vasodilation.10) Similarly, Tsai et al.11) demonstrated that elevated plasma viscosity increased perivascular NO production in concert with the increased aortic eNOS protein expression during extreme hemodilution. Studies in awake animals treated with high viscosity plasma expanders (HVPEs) showed advantageous effects in the microvascular function in both hemorrhagic shock-resuscitation and acute extreme hemodilution models.12)13)14) These studies revealed that an elevated plasma viscosity positively correlates with increased WSS and NOS. The vascular network connected with the heart is a complex biological coupling. Therefore, the effects of increased plasma viscosity by plasma expanders (PEs) on the circulatory system are being continually investigated in order to gain a better understanding related to novel PE development. Recently, using a miniature pressure-volume (PV) conductance catheter in an acute hemodilution, it was found that HVPE provides better effects on cardiac function compared with low viscosity plasma expanders (LVPE).15) Furthermore, a recent study has demonstrated that animals hemodiluted with LVPE combined with a proper amount of NO donor had positive effects on cardiac function, which could not be observed in animals hemodiluted with LVPE only.16) The aim of this study was to assess the response of cardiac function to NOS inhibitors during an elevated plasma viscosity but with low Hct level.
The experiments were carried out in anesthetized male Golden Syrian hamsters with a weight between 60 and 70 g (Charles River Laboratories; Boston, MA, USA). The animal handling and care followed the NIH Guideline for Care and Use of Laboratory Animals. The experimental study was approved by the animal care committee of the University of California, San Diego. Animal surgery was performed following an intraperitoneal (i.p.) administration of sodium pentobarbital (50 mg/kg). The left jugular vein was cannulated to allow infusion fluid and the left femoral artery was catheterized for systemic blood pressure monitoring and blood withdrawal and sampling. In addition, a tracheotomy was conducted and the animal was cannulated with a polyethylene-90 tube to facilitate spontaneous breathing. The body core temperature was maintained at around 37℃ by being placed in the supine position on a heating pad. During the experiment, if the animals responded to a toe pinching, a small bolus of sodium pentobarbital (10.15 mg/kg, i.p.) was administered for maintaining an anesthesia condition.
Animals under anesthesia were included in the experiments if they had no signs of bleeding and their systemic parameters were within the normal range: 1) mean arterial pressure (MAP) above 80 mm Hg, 2) heart rate (HR) above 320 beats/minute, and 3) systemic Hct level above 45%.
An isovolemic hemodilution was performed using PE consisting of a solution of Dextran 6% T2000 (Pharmacosmos, Holbaek, Denmark) in 0.9% sodium chloride mixed with 10% human serum albumin. This procedure has been reported elsewhere, briefly, forty percent (40%) of the animals blood volume (BV) estimated as 7% of body weight was withdrawn from the femoral artery catheter using a dual syringe pump (33 syringe pump, Harvard Apparatus, Holliston, MA, USA) at a rate of 100 µL/min.17) Simultaneously, PE was administered into the jugular vein catheter at the same rate to lower the systemic Hct to about 20%. The experimental protocol is schematically shown in Fig. 1. The animals were monitored for 1 hour after the completion of the hemodilution. At the end of experiment, blood samples were collected to measure plasma viscosity, plasma colloid osmotic pressure (COP) and blood conductance. The PE solution had a viscosity of 6.3 cP and 43 mm Hg in COP.
Ten animals were entered into the study and randomly assigned to two groups. Animals in the first group (n=5) underwent hemodilution with only the PE (Control Group). Animals in the second group (n=5) were administered with 10 mg/kg of L-NAME (i.v.) 20 minutes before being hemodiluted with the PE (Treated Group). This dose of L-NAME was a low dose as reported by Sakai et al.8)
The MAP was continuously monitored using a fluid-filled pressure transducer and a signal acquisition system (MP150, Biopac System Inc., Santa Barbara, CA, USA). The systemic Hct level was determined from arterial blood samples taken in heparinized capillary tubes and centrifuged. Viscosity was measured at a shear rate of 160s-1 using a cone and plate viscometer (Brookfield Engineering Laboratories, Middleboro, MA, USA). The COP of PE and blood plasma was measured using a membrane colloid osmometer (model 420, Wescor, Logan, UT, USA).
The closed-chest method was used to assess cardiac function.18) Briefly, the right common carotid artery was exposed allowing to insert a 1.4 Fr PV conductance catheter (PV catheter; SPR-839, Millar Instruments, Houston, TX, USA). The PV catheter was advanced passing through the aortic valve into the left ventricle. At baseline conditions and the end of the experiment, a small bolus (10 µL) of 15% hypertonic saline was administered intravenously to determine the parallel volume. The left ventricular (LV) pressure and volume measurements were digitized and acquired at 1 kHz sampling rate (MPVS300, Millar Instruments, Houston, TX, USA and PowerLab 8/30, ADInstruments, Colorado Springs, CO, USA). Then the cardiac function data was analyzed with PVAN software (version 3.6, Millar Instruments, Houston, TX, USA). The selected 8-12 cardiac cycles were analyzed for cardiac function indices at each time point.
The LV BV was measured in conductance units (relative volume unit) and converted to actual BV (µL) using a known-volume cuvette at the end of the experiment. The blood conductance at baseline was estimated from the baseline Hct level, using the pooling data of blood conductance and Hct relationship.17)
The results are depicted as mean±standard deviation. The data pertaining to the same group were evaluated using the analysis of variance and Dunnett's multiple comparison test for post hoc analyses. An unpaired, two-tailed t-test was performed to compare between groups at each time point.
All of the statistics were performed using GraphPad Prism 5.04 (GraphPad Software, San Diego, CA, USA). Statistical significance was determined at a p<0.05.
The MAP (Fig. 2A) increased 30% from baseline to 20 minutes after L-NAME administration. The effect was long lasting, for at least 30 minutes after hemodilution, and returned closer to baseline levels at 60 minutes after hemodilution. In contrast, the MAP in the control group was at all points significantly lower after hemodilution than at baseline and was significantly lower than in the treated group where it was only at time point 15 minutes after hemodilution. The HRs (Fig. 2B) in the treated group decreased by 15% from the baseline, mainly after hemodilution. In the control group, the HR remained near baseline values after hemodilution. The systemic Hct level decreased from 53±2% to 27±2% and 53±4% to 25±2% in the control group and the L-NAME-treated group, respectively. Table 1 summarizes the systematic parameters in each group at the different time points. Blood viscosity in the control group after hemodilution was 3.61±0.24 cP whereas plasma viscosity was 2.12±0.10 cP. These viscosities were not significantly different compared to that of the treated group.
Fig. 3A shows the end-systolic pressure (Pes) and end-diastolic pressure (Ped) at baseline and 15 minutes and 60 minutes after hemodilution, Pes significantly increased after L-NAME injection (25% higher than the baseline) and remained higher after hemodilution compared to the baseline, whereas Ped did not change after L-NAME injection. For the control group Pes was significantly lower than baseline for all times after hemodilution; Ped remained around baseline values. Fig. 3B shows an example of LV pressure and volume curves for the control group and the treated group at three time points of interest (baseline, 15 minutes and 60 minutes after hemodilution). There was no difference between both groups for both LV pressure and volume at baseline. Animals treated with L-NAME had a higher LV pressure than the animals hemodiluted with HVPE only, especially at 15 minutes after hemodilution. Fig. 3C shows the maximum and minimum rates of pressure change (dP/dt) for the same points of interests. The maximum rate of pressure rise (+dP/dtmax) decreased after hemodilution in both groups when compared to the baseline. In the L-NAME treated group, +dP/dtmax was significantly lower than in the control group where it was only 60 minutes after hemodilution. As shown in Fig. 3C, the maximum rate of pressure fall (-dP/dtmax) in the L-NAME group was significantly higher than in the control group (p<0.05) for every time point after hemodilution. These parameters were also summarized in Table 1 at the time points of interest.
Fig. 4 shows the cardiac function indices for the control and treated groups. Cardiac output (CO) was significantly decreased in the treated group after L-NAME injection at all-time points compared to both, baseline and control group. Stroke volume (SV) was significantly decreased after hemodilution compared to the control group, but remained without significant changes compared to baseline. Stroke work (SW) remained with no significant changes between groups and relative to baseline up to 15 minutes after hemodilution. However, it was significantly increased in the control group at 30 minutes and 60 minutes after hemodilution, at this last time point it was significantly higher (p<0.05) for the control group compared to the treated group. The ejection fraction was not statistically different between groups or compared to baseline. However, it exhibited a trend to decrease (by 14%) in the treated group after L-NAME injection and to increase in the control group during hemodilution.
As L-NAME significantly increased MAP and reduced CO, the treated group presented a higher systemic vascular resistance (SVR) compared to the control group (p<0.05) as presented in Fig. 5. The SVR in the treated group was significantly higher than at the baseline (by 35%), whereas SVR in the control group dropped 32% below the baseline (p<0.05) after hemodilution. Fig. 6 illustrates examples of PV loops during the period of the experiment (baseline, after L-NAME administration and 15 minutes after hemodilution). The PV loop in the control group shifted to the right after hemodilution, indicating an increase in cardiac preload. In contrast, the PV loops in the L-NAME group did not show any right shift, but there was an apparent increase in Pes due to the effect of L-NAME.
This study demonstrated that eNOS inhibition prevents the normal cardiac adaptive response after anemic states. Using HVPE in an isovolemic hemodilution to elevate plasma viscosity provided a positive cardiac adaptation to the lowered oxygen carrying capacity of blood, but the eNOS inhibition reflected negative effects on the load dependent cardiac indices after hemodilution. This study supports the view that NO production plays an important role in the regulation of cardiac performance during hemodilution when induced by shear stress on endothelium from elevated plasma viscosity.
In previous studies, it was demonstrated that the PE viscosity plays a major role in the maintenance of microcirculatory function and cardiac performance during anemic states.13)15) In this study, however, it was shown that the positive effects of HVPE use are weakened by the inhibition of eNOS. This effect may be explained by the relationship between endothelial WSS and the endothelial mediated NO production which is similar to the work demonstrated by Tsai et al.11) In normal conditions, the production of NO by the endothelium is mediated by WSS.
In the present study, before hemodilution, both high mean arterial blood pressure and high Pes were clearly observed in animals intravenously injected with L-NAME, indicating there was an increase in afterload. During the first 30 minutes after L-NAME infusion, it was observed that L-NAME caused a significant change in mean arterial blood pressure due to vasoconstriction induced by the eNOS-inhibitory effect of L-NAME. However, this effect was not prolonging. Furthermore, the SVR after L-NAME administration significantly increased, causing the heart to increase pumping pressure to overcome this resistance, and remained higher than the baseline even after hemodilution. Similarly, Sakai et al.8) observed that the inhibition of NOS activities by L-NAME induced systemic hypertension, constriction of resistant arteries and reduction of microvascular blood flow. Furthermore, several previous studies have shown that the inhibition of NOS causes coronary vasoconstriction in both isolated hearts and conscious animals.19)20)21) The NOS inhibitors are guanidino amino acids, which compete with binding molecules at the NOS active site. L-NAME is a non-selective NOS inhibitor, which can inhibit the constitutive isoform of NOS, including eNOS. Therefore, this inhibition can negatively affect the vascular tone regulation. Although animals treated with L-NAME were hemodiluted with HVPE, the mean arterial blood pressure as well as the Pes was higher compared with those of animals without treatment and hemodiluted with HVPE. This indicates that induced-shear stress NO can not be produced, leading to a vasoconstrictive effect in the animals treated with L-NAME.
Animals with L-NAME treatment showed a significant simultaneous decrease in the HR after L-NAME infusion. As treated animals were hemodiluted with HVPE, a continuous drop in HR was observed. In contrast, animals without L-NAME treatment, demonstrated a slight decrease in HR after hemodilution. With the study by Pabla and Curtis20) on the effects of NO modulation on cardiac arrhythmias in isolated rat hearts it was reported that L-NAME caused sinus bradycardia-similar to the results of other in vivo studies reporting on the bradycardia effects of NOS.22)23) Recently, NO donor administration in animals hemodiluted with Dextran 70 kDa demonstrated the chronological effect of a 4% increase in the HR from the baseline, which was significantly increased when compared to animals without NO donor administration.16) In addition, Bryan et al.24) have reported the effects on hemodynamics in a normal Hct level, that the pre-administration of S-nitro-N-acetylpenicillamine followed by L-NAME administration and vice versa can decrease systolic blood pressure and mean arterial blood pressure, while the HR is increased.25) These previous studies emphasize the role of NO on HR. Therefore, it is important to note that the inhibition of NOS or the decrease in NO production coupled with hemodilution with HVPE can cause a negative effect on HR. However, controversial and unclear issues related to the chronological effects of NO still remain, especially in in vivo studies.26)
In the present study, the CO markedly decreased after L-NAME infusion followed by hemodilution with HVPE, while CO in the group without L-NAME treatment significantly increased from the baseline. This finding is in agreement with those of several studies, which have indicated that L-NAME decreases the CO in a normal level of Hct either in the short-term or long-term treatments with L-NAME.25)27)28) Furthermore, in this study, the L-NAME administration led to a reduction in SV even after hemodilution. Especially at 15 minutes after hemodilution as shown by PV loops, it could be noticed that the end-diastolic volume decreased after hemodilution in animals with L-NAME treatment which led to lower SV. Generally, there is a compensatory mechanism for a reduction in oxygen transport capability. i.e., hemodilution by an increase in CO via either an increase in HR or an enhancement of SV. This compensation was still observed after hemodilution in animals without L-NAME treatment but not in animals treated with L-NAME. By administration through a coronary catheter, using non-selective NOS inhibitors such as N-nitro-L-arginine (L-NNA) in normal dogs an increment in the coronary blood flow was shown.29) In contrast, Biwer et al.28) demonstrated that long-term L-NAME-treated rats had not only a decrease in CO and SW but also a decrease in coronary blood flow, which potentially caused cardiac dysfunction. Therefore, it is a point of concern that using PEs in hemodilution with a stage of NOS impairment can lead to an attenuation of the cardiac compensatory mechanism.
The use of a non-selective NOS inhibitor, L-NNA, resulted in an increase in myocardium oxygen consumption in normal un-anesthetized dogs, whereas using L-NAME in anesthetized open-chest dogs did not present this.29)30) In the present study, after hemodilution with HVPE, animals treated with L-NAME presented a lower SW and +dP/dtmax than animals without L-NAME treatment. This indicates an attenuation of energy to pump out blood from the chamber. However, this attenuation of energy related to a decrease in SV and a smaller PV loop area. Therefore, it can be pointed out that an impairment of NOS significantly affects cardiac load-dependent indices after hemodilution. Also the heart seems to be less able to adjust to the change of reduced Hct content in animals treated with L-NAME.
The analogs of L-arginine were used to treat some patient's conditions such as hypotension during septic shock. As a result of the presented study, it was demonstrated that the cardiac compensatory mechanism attenuated by L-NAME responded to hemodilution. Therefore, it might not be beneficial to administer L-NAME in patients with low Hct and septic shock or with a required hemodilution regarding to the modalities of sepsis treatment. On the other hand, for septic patients with L-NAME administration a high viscosity PE might not be suitable for volume replacement. However, in the present study L-NAME administration was performed as a short-term treatment and therefore it is necessary to look at longterm treatment effects on a hemodiluted condition. Furthermore, the study had several limiting issues such as a small number of animals in each group, only two dosages of L-NAME, no oxygen delivery assessment and the effects of anesthetics (sodium pentobarbital) as a cardiac depressor.
In conclusion, it was shown that eNOS inhibition prevents the normal cardiac adaptive response after anemic states. Using high viscogenic PE in isovolemic hemodilution to elevate plasma viscosity provided a positive cardiac adaptation to the lowered oxygen carrying capacity of blood, but eNOS inhibition reflected negative effects on load dependent cardiac indices.
Figures and Tables
Fig. 1
Schematic diagram of acute isovolemic hemodilution protocol for animals treated with L-NAME. BL: baseline, TM: treatment, HD15: hemodilution 15 minutes, HD30: hemodilution 30 minutes, HD60: hemodilution 60 minutes, L-NAME: N(G)-nitro-L-arginine methyl ester.
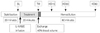
Fig. 2
Mean arterial blood pressure (MAP) (A) and heart rate (HR) (B) measured at baseline (BL), after N(G)-nitro-L-arginine methyl ester treatment (TM), at 0, 15, 30, and 60 minutes after hemodilution (HD). *p<0.05 compared to BL, †p<0.05 compared between groups.
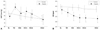
Fig. 3
Left ventricular (LV) end-systolic pressure (Pes) and LV end-diastolic pressure (Ped) measured at baseline (BL), after N(G)-nitro-L-arginine methyl ester (L-NAME) treatment (TM), at 15, 30, and 60 minutes after hemodilution (HD) (A). Example of LV pressure and volume waveforms at BL, after L-NAME TM and at 60 minutes after HD (B). Maximum rate of pressure rise (+dP/dtmax) (C) and Maximum rate of pressure fall (-dP/dtmax) (B) recorded at BL, after L-NAME TM, at 15, 30, and 60 minutes after HD. Values are relative to baseline and presented as mean±SD. *p<0.05 compared to BL, †p<0.05 compared between groups.
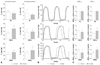
Fig. 4
Cardiac function indices such as cardiac output (CO) (A), stroke volume (SV) (B), stroke work (SW) (C), and ejection fraction (EF) (D) present at baseline (BL), after N(G)-nitro-L-arginine methyl ester treatment (TM), at 0, 15, 30, and 60 minutes after hemodilution (HD). Values are relative to BL and presented as means±SD. *p<0.05 compared to BL, †p<0.05 compared between groups.
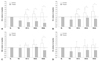
Fig. 5
Systemic vascular resistance (SVR) at baseline (BL), after N(G)-nitro-L-arginine methyl ester treatment (TM), at 15, 30, and 60 minutes after hemodilution (HD). Values are relative to BL and presented as mean±SD.
*p<0.05 compared to BL, †p<0.05 compared between groups.
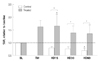
Fig. 6
Examples of pressure-volume loops in the control group and the L-NAME-treated group at baseline (BL), after L-NAME treatment (TM) and at 15 minutes after hemodilution (HD15). LV: left ventricular, L-NAME: N(G)-nitro-L-arginine methyl ester.
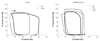
Table 1
Systemic parameters and pressured related cardiac function measured at BL, after L-NAME TM, at 15, 30, and 60 minutes after HD
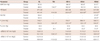
Acknowledgments
The authors greatly thank Cynthia Walser for the surgical preparation of the animals. This work was supported by the Bioengineering Research Partnership grant R24-HL64395, program project P01-HL071064, grant R01-HL62354 and R01-HL62318 and by the Faculty of Medicine, Prince of Songkla University grant 54-221-25-2-3.
References
1. Boo YC. Shear stress stimulates phosphorylation of protein kinase A substrate proteins including endothelial nitric oxide synthase in endothelial cells. Exp Mol Med. 2006; 38:453.
2. Corson MA, James NL, Latta SE, Nerem RM, Berk BC, Harrison DG. Phosphorylation of endothelial nitric oxide synthase in response to fluid shear stress. Circ Res. 1996; 79:984–991.
3. Shesely EG, Maeda N, Kim HS, et al. Elevated blood pressures in mice lacking endothelial nitric oxide synthase. Proc Natl Acad Sci U S A. 1996; 93:13176–13181.
4. Rastaldo R, Pagliaro P, Cappello S, et al. Nitric oxide and cardiac function. Life Sci. 2007; 81:779–793.
5. Palmer RM, Rees DD, Ashton DS, Moncada S. L-arginine is the physiological precursor for the formation of nitric oxide in endothelium-dependent relaxation. Biochem Biophys Res Commun. 1988; 153:1251–1256.
6. Hsieh NK, Chang HR, Hu CT, Chen HI. Effects of nitric oxide donor and nitric oxide synthase inhibitor on the resistance, exchange and capacitance functions of the canine intestinal vasculature. Vascul Pharmacol. 2008; 48:122–128.
7. Fitch RM, Vergona R, Sullivan ME, Wang YX. Nitric oxide synthase inhibition increases aortic stiffness measured by pulse wave velocity in rats. Cardiovasc Res. 2001; 51:351–358.
8. Sakai H, Hara H, Tsai AG, Tsuchida E, Intaglietta M. Constriction of resistance arteries determines l-NAME-induced hypertension in a conscious hamster model. Microvasc Res. 2000; 60:21–27.
9. Fozard JR, Part ML. Haemodynamic responses to NG-monomethyl-L-arginine in spontaneously hypertensive and normotensive Wistar-Kyoto rats. Br J Pharmacol. 1991; 102:823–826.
10. de Wit C, Schäfer C, von Bismarck P, Bolz SS, Pohl U. Elevation of plasma viscosity induces sustained NO-mediated dilation in the hamster cremaster microcirculation in vivo. Pflugers Arch. 1997; 434:354–361.
11. Tsai AG, Acero C, Nance PR, et al. Elevated plasma viscosity in extreme hemodilution increases perivascular nitric oxide concentration and microvascular perfusion. Am J Physiol Heart Circ Physiol. 2005; 288:H1730–H1739.
12. Cabrales P, Intaglietta M, Tsai AG. Increase plasma viscosity sustains microcirculation after resuscitation from hemorrhagic shock and continuous bleeding. Shock. 2005; 23:549–555.
13. Cabrales P, Tsai AG. Plasma viscosity regulates systemic and microvascular perfusion during acute extreme anemic conditions. Am J Physiol Heart Circ Physiol. 2006; 291:H2445–H2452.
14. Cabrales P, Tsai AG, Intaglietta M. Increased plasma viscosity prolongs microhemodynamic conditions during small volume resuscitation from hemorrhagic shock. Resuscitation. 2008; 77:379–386.
15. Chatpun S, Cabrales P. Cardiac mechanoenergetic cost of elevated plasma viscosity after moderate hemodilution. Biorheology. 2010; 47:225–237.
16. Chatpun S, Cabrales P. Exogenous intravascular nitric oxide enhances ventricular function after hemodilution with plasma expander. Life Sci. 2012; 90:39–46.
17. Chatpun S, Cabrales P. Effects of plasma viscosity modulation on cardiac function during moderate hemodilution. Asian J Transfus Sci. 2010; 4:102–108.
18. Pacher P, Nagayama T, Mukhopadhyay P, Bátkai S, Kass DA. Measurement of cardiac function using pressure-volume conductance catheter technique in mice and rats. Nat Protoc. 2008; 3:1422–1434.
19. Amezcua JL, Palmer RM, de Souza BM, Moncada S. Nitric oxide synthesized from L-arginine regulates vascular tone in the coronary circulation of the rabbit. Br J Pharmacol. 1989; 97:1119–1124.
20. Pabla R, Curtis MJ. Effects of NO modulation on cardiac arrhythmias in the rat isolated heart. Circ Res. 1995; 77:984–992.
21. Rees DD, Palmer RM, Schulz R, Hodson HF, Moncada S. Characterization of three inhibitors of endothelial nitric oxide synthase in vitro and in vivo. Br J Pharmacol. 1990; 101:746–752.
22. Pfeiffer S, Leopold E, Schmidt K, Brunner F, Mayer B. Inhibition of nitric oxide synthesis by NG-nitro-L-arginine methyl ester (L-NAME): requirement for bioactivation to the free acid, NG-nitro-L-arginine. Br J Pharmacol. 1996; 118:1433–1440.
23. Ward JE, Angus JA. Acute and chronic inhibition of nitric oxide synthase in conscious rabbits: role of nitric oxide in the control of vascular tone. J Cardiovasc Pharmacol. 1993; 21:804–814.
24. Bryan S, Alexander-Lindo R, McGrowder D. The effect of nitric oxide inhibitors and s-nitrosothiols on hemodynamic parameters in an animal model. Open Access Animal Physiology. 2011; 3:1–8.
25. Crystal GJ, Zhou X, Halim AA, Alam S, El-Orbany M, Salem MR. Nitric oxide does not modulate whole body oxygen consumption in anesthetized dogs. J Appl Physiol (1985). 1999; 86:1944–1949.
26. Herring N, Paterson DJ. Endothelial nitric oxide synthase and heart rate. Circulation. 2002; 106:e5. author reply e5.
27. Widdop RE, Gardiner SM, Kemp PA, Bennett T. The influence of atropine and atenolol on the cardiac haemodynamic effects of NG-nitro-L-arginine methyl ester in conscious, Long Evans rats. Br J Pharmacol. 1992; 105:653–656.
28. Biwer LA, Broderick TL, Xu H, Carroll C, Hale TM. Protection against L-NAME-induced reduction in cardiac output persists even after cessation of angiotensin-converting enzyme inhibitor treatment. Acta Physiol (Oxf). 2013; 207:156–165.
29. Chen Y, Traverse JH, Du R, Hou M, Bache RJ. Nitric oxide modulates myocardial oxygen consumption in the failing heart. Circulation. 2002; 106:273–279.
30. Crystal GJ, Zhou X. Nitric oxide does not modulate the increases in blood flow, O2 consumption, or contractility during CaCl2 administration in canine hearts. Cardiovasc Res. 1999; 42:232–239.