Abstract
Background and Objectives
The heat-shock response modulates contractility of vascular smooth muscles. With complementary deoxyribonucleic acid microarray, we tried to identify the novel genes that are involved in the regulation of vascular contraction after heat shock.
Materials and Methods
Human radial artery strips were mounted in organ baths, exposed at 42℃ for 45 minutes, and returned to equilibrate at 37℃. This study examined gene expression profile associated with heat-shock response in radial arteries of patients with hyperlipidemia by using a microarray that contained 5763 human cDNA. The results of microarray hybridization experiments from the radial arteries of 4 different subjects were analyzed and classified by the cluster program.
Results
Among these differentially-expressed genes, Hsp70, Hsp10, αB-crystallin, and Hsp60 were significantly increased by the heat shock response. Of non-HSP genes, 15 genes increased, while 22 genes decreased. Among these 37 genes, αB-crystallin (CRYAB) (up 1.92-fold), myosin, light polypeptide kinase transcript variant 8, 6 (up 1.70-fold, up 1.68-fold), catenin (cadherin-associated protein, alpha-like 1) (down-0.57 fold) and tropomyosin 3 (down 0.68-fold) were thought to be related with the contraction. Real-time quantitative polymerase chain reaction showed that Hsp70, Hsp10 and αB-crystallin were significantly increased.
Stress proteins have been implicated in pathophysiologic cardiovascular conditions such as chronic hypertension and atherosclerosis.1) Also, hyperlipidemia represents a determinant for the development of atherosclerosis and an important risk factor for cardiovascular disease.2) Although the acute stress response is a well-known mechanism of restoring homeostasis after cellular stresses,3) it has been suggested that chronic expression of heat-shock proteins (HSPs) is deleterious.4) Because the stress response affects the expression pattern of numerous genes, the comparison of the expression profile of genes between control and heat shock groups of radial arteries may provide information about the discovery of novel proteins to regulate contraction. Therefore, to further understand the molecular basis of heat shock response, it would be of interest to identify and to characterize differentially expressed genes. The complementary deoxyribonucleic acid (cDNA) microarray method has an advantage to monitor the expression of hundreds or thousands genes simultaneously.5) In this study, in order to identify the genes that are involved in the heat shock response of human radial arteries, we analyzed the gene expression profiles of 4 human radial artery samples using a KNU Human 5.8 K cDNA chip.6)
Human cDNA clones were obtained from The Human Stromal Cells cDNA Bank at the Hair Research Center, the Kyungpook National University, Daegu, Korea. The cDNA libraries were made from human dermal papilla cells and per follicular sheath cells. The polymerase chain reaction (PCR)-amplified cDNAs were printed onto Amino-silane coated glass slides (CMT-GAPS™ coated slides) (Corning Inc.) using a robotic transfer device (Cartesian PixSYS 5500). A total 5763 cDNAs, consisting of 5571 human clones and 192 controls (64 spots each of glyceraldehyde-3-phosphate dehydrogenase, beta-actin and alpha-tubulin as intraslide controls), were arrayed in 1.8×1.8 cm areas. Hybridization data was considered invalid if the intensity of the same control gene showed more than 1.5-fold or less than 0.7-fold difference.
We obtained informed consent from 4 patients, who had undergone isograft bypass surgery. The remnants of the bypass surgeries were used in our studies. All 4 patients were males with a history hyperlipidemia and smoking >30 years. The clinical characteristics of patients donating radial arteries are shown in Table 1.
Each arterial tissue from the 4 patients was divided into 2 segments (control and heat-shock groups). After equilibration at 37℃ for at least 1 hour, heat-shock group were exposed to 42℃ for 45 minutes and returned to equilibrate at 37℃, whereas the other control group was left at 37℃. Both of the groups were further incubated for 4 hours until they were frozen with liquid nitrogen and stored at -80℃ for subsequent microarray and reverse transcriptase-quantitative polymerase chain reaction (RT-qPCR) experiments.
Human radial arteries were homogenized in liquid nitrogen until they looked like powder. Ribonucleic acid (RNA) was extracted in 2 mL of TRI Reagent (Molecular Research Center, Cincinnati, OH, USA) according to the manufacturer's protocol. RNA concentration was determined with a spectrophotometer. Messenger RNA was isolated from total RNA using a Dynabead mRNA purification kit (Dynal). In order to obtain sufficient RNA for the analysis, we amplified total RNA by in vitro transcription using Ampliscribe T7 Transcription Kits (Epicenter Technologies) as described before.7) By reverse transcription, amplified RNAs from both the normal and heat-shocked radial artery were labeled with Cy3- and Cy5-dUTP (Amersham), respectively, as described previously.7) For prehybridization, we incubated the slides in 3.5 X SSC, 0.1% sodium dodecyl sulfate (SDS), and 10 mg/mL bovine serum albumin in a Coplin jar for 20 minutes at 50℃, then washed in water and isopropanol by dipping. Next, the slides were then dried by centrifugation at 500 rpm for 5 minutes. Following incubation with probes at 99℃ for 2 minutes, the slides were placed in a Corning CMT-Hybridization chamber for 20 hours at 50℃. The slides were washed in a low-stringency wash buffer for (0.1X SSC/0.1% SDS) 5 minutes, then in a high-stringency wash buffer (0.1X SSC) for 5 minutes, and dried by centrifugation.
Fluorescence intensities were measured using a scanarray 4000 with a laser confocal microscope (GSI Group Inc., Bedford, MA, USA). The 2 fluorescent images (Cy3 and Cy5) were scanned separately, and the color images were formed by arbitrarily assigning the heat shock intensity values and background intensity into red and green channels, respectively. The data were analyzed using Quantarray software (version 2.0.1, GSI Lumonics). The results were analyzed by normalization between the images to adjust for the different efficiencies in labeling and detection between the two different fluorescences. We used a filter so that all of the genes exhibited a level of expression intensity of more than 3000 fluorescent units (on a scale of 0-65535 fluorescent units) for both red and green channels for each experiment. Data from the hybridization experiment were viewed as a normalized ratio (that is, Cy5/Cy3).
To analyze the results of the differentially expressed genes, we performed background intensity subtraction, data normalization, and clustering. With the use of image analysis software, the signal intensities were determined for each dye at each element of the array, and the ratio of Cy5 intensity to Cy3 intensity was calculated. The measured Cy5/Cy3 fluorescence ratio values were logarithmatically transformed (base 2 for simplicity) in order to easily quantify inductions or repressions of identical magnitude, as numerically equal but with an opposite sign.
Total RNA (2 µg) was reverse-transcribed into cDNA by using RevertAid™ first strand cDNA synthesis (Thermo Fisher Scientific, Glen Burnie, MD, USA) in 20 µL reaction volume according to manufacturer's instructions. Real-time PCR was performed using ABI Prism 7000 sequence detection system (Applied Biosystems, Foster City, CA, USA). Ten microliters of SYBR Green PCR master mix (Takara Bio Inc., Otsu, Shiga, Japan), 4 µL of cDNA, and 200 nmol/L primer set were used for amplification in a 20 µL reaction volume. All samples were amplified in triplicates in a 96-well plate and the cycling conditions were as follows: 2 minutes at 50℃, 10 minutes at 95℃, and 40 cycles at 95℃ for 15 seconds followed by 1 minute at 60℃. The relative mRNA expression level was determined by calculating the values of Δcycle threshold (ΔCt) by normalizing the average Ct value compared with its endogenous control (Gapdh) and then calculating 2-ΔΔCt values.1) All primer sets used in real-time PCR are shown in Table 2.
Among the total 5.763 cDNA clones, 15 cDNA elements were upregulated (Cy5/Cy3 >1.5) and 22 elements were down-regulated (Cy5/Cy3 <0.7).
As shown in Table 3, four HSPs such as Hsp70, Hsp10, crystalline-αB and Hsp60 were significantly upregulated. Among non-HSPs genes, 14 upregulated genes were listed in Table 4, whereas 22 down-regulated genes were listed in Table 5. Table 4 and 5 had the genes such as myosin, light polypeptide kinase (MYLK), transcript variant 8 (up 1.70-fold), MYLK, transcript variant 6 (up 1.68-fold), armadillo repeat protein ALEX2 (ALEX2) (down 0.56-fold), catenin (cadherin-associated protein), alpha-like 1 (CTNNAL1) (down 0.57-fold) and tropomyosin 3 (TPM3) (down 0.68-fold).
The genes related to the vascular contraction were classified as a new group (Table 6). We expected potassium large conductance calcium-activated channel, subfamily M, beta member 1 (KCNMB1), calmodulin 1 (phosphorylase kinase, delta) (CALM1), actinin, alpha 1 (ACTN1), thymosin, beta 4, X-linked (TMSB4X), dynein, cytoplasmic, light polypeptide 1 (DNCL1), myosin regulatory light chain MRCL3 (MRCL3), cofilin 2 (muscle) (CFL2), transcript variant 2, tropomyosin 1 (alpha) (TPM1), Rho/Rac guanine nucleotide exchange factor (GEF) 2 (ARHGEF2), Rho GDP dissociation inhibitor (GDI) alpha (ARHGDIA) and Rho GTPase activating protein 18 (ARHGAP18) genes to be increased, but there was found no significant change found on them.
Four HSPs such as Hsp70, Hsp10, crystalline-αB and Hsp60 shown in Table 3 were validated by quantitative real-time PCR. The genes Hsp70, Hsp10, and crystalline-αB were significantly upregulated.
Heat-shock proteins are synthesized by cells subsequent to a stress exposure and are known to confer protection to the cell in response to a second challenge. HSP induction and decay are correlated to thermotolerance and may therefore be used as a biomarker of thermal history. As shown in Table 3, the significant change in expression was found at least in one representative member of most major families of known human HSP. As might be expected from a heat-induced process, only 3 HSPs were significantly upregulated. The induction of Hsp70 associated with acute hypertension in the rat aorta has been suggested to help protect the vasculature from damage during hemodynamic stress,9) whereas chronic expression of Hsp70 has been implicated in hypertension in animal studies.10) Mammalian heat shock protein 10 (Hsp10) and heat shock protein 60 (Hsp60), also known as chaperonins 10 and 60, are mitochondrial proteins involved in protein folding. In mitochondria, Hsp10 forms a heptameric lid, which binds to a double-ring toroidal structure comprising seven Hsp60 subunits per ring.11) Anti-Hsp60 antibodies prevented and mimicked Hsp10 inhibitory activity, suggesting that Hsp10 may inhibit pro-inflammatory responses by interacting with extracellular Hsp60.12)13)
Heat-shock proteins exported to the plasma membrane or released from dying cells are believed to be a source of "danger" signals, informing the innate and adaptive immune systems of tissue damage induced by various insults including infection, injury, toxins, heat, and/or cellular stress.14)15)
The non-HSPs genes strongly affected by heat-shock were listed in Table 2 and 3. Importantly, 15 upregulated genes were listed in Table 4, while 22 down-regulated genes in Table 5. Table 4 and 5 had genes, such as crystallin, alpha B (CRYAB) (up 1.92-fold), MYLK, transcript variant 8 (up 1.70-fold), MYLK, transcript variant 6 (up 1.68-fold), armadillo repeat protein ALEX2 (down 0.56-fold), CTNNAL1 (down 0.57-fold) and TPM3 (down 0.68-fold). It would be interesting that these genes were related with the vascular contraction. Therefore, these genes were classified as a new group (Table 6) including some expression genes, which were not statistically significant. We expected potassium large KCNMB1, CALM1, ACTN1, TMSB4X, DNCL1, MRCL3, CFL2, TPM1, ARHGEF2, ARHGDIA and ARHGAP18 genes to be increased but it was found no significant change on them. Our laboratory reported that direct heat shock of rat isolated aorta at 42℃ for 45 minutes augmented its contractility several hours later.16) However, these regulated genes have not been characterized with respect to contractile functions so far.
The major structural protein, αB-crystallin, a member of the small heat-shock protein (sHSP) family, was identified originally in ocular lens tissue.17) αB-Crystallin has several tissue-specific roles. In addition to facilitating light refraction in the lens, it assists protein folding in vivo and in vitro.18) αB-Crystallin may be important for nascent myosin folding, myofibrillogenesis, cytoskeleton integrity, and maintaining muscle performance during stress conditions.
Myosin is the molecular motor that drives actomyosin-based motility, including muscle shortening and cytokinesis, by ATP-dependent conformational changes.19) Thermally and chemically induced unfolding of myosin in vitro appears irreversible, and it has been proposed that chaperones are required for proper folding of the motor.20)21) αB-crystallin interacts with unfolded myosin dimers and filamentous bundles to prevent misfolding under stress. Therefore, αB-crystallin may be useful in highlighting the importance of chaperones in maintaining the contractile machinery as well as the cytoskeleton.
Myosin, light polypeptide kinase gene, a muscle member of the immunoglobulin gene superfamily, encodes myosin light chain kinase which is a calcium/calmodulin dependent enzyme. This kinase phosphorylates myosin regulatory light chains to facilitate myosin interaction with actin filaments to produce contractile activity. The C-terminal regions of both smooth muscle and nonmuscle MLCK isoforms contain a C2-type immunoglobulin-like domain known as kinase-related protein or telokin. This region facilitates binding of the enzyme to the unphosphorylated MLC or myosin and consequently promotes MLCK contractile activities.22)23)
Tropomyosin (TM), an actin binding protein and a major component of the sarcomeric thin filament, forms coiled-coil dimers that assemble in a head-to-tail fashion in the major groove of actin. Binding of Tm to F-actin is influenced by intrinsic interactions between Tm and actin monomers, interactions between overlapping head-to-tail regions of contiguous Tm molecules along the actin filament, and by other proteins such as Tn and myosin that greatly increase the binding. The TM gene family is composed of four genes {α-TM, β-TM, TPM 3 (γ-TM), and TPM 4 (δ-TM)}, which through alternative splicing, the use of alternative promoters, and differential processing encode multiple striated muscle, smooth muscle, and nonmuscle specific transcripts.24)25) TM, along with the troponin complex, regulates the Ca2+- sensitive reaction of cross bridges with actin in striated musculature. However, smooth muscle contains no troponin, thin filaments contain only actin and tropomyosin.26) Therefore, the decrease in TM3 by heat shock resulted in the increase of myosin-actin interaction and stimulated muscle contraction.
Contractile stimulation has been shown to initiate actin polymerization in smooth muscle tissues, and this actin polymerization is required for active tension development. Recent studies have provided evidence that contractile stimulation induces the polymerization of F-actin from a pool of G-actin in smooth muscle tissues.27)28) The CTNNAL1 gene shows extensive homology to a-catenin that was found to be ubiquitously expressed in many tissues including pancreas, heart, and skeletal muscle. It was found that α-catenin suppressed Arp2/3-mediated actin polymerization by directly competing with Arp2/3 for actin filaments.29) Therefore, the results showed the significant decrease of α-catenin by heat shock could not block actin polymerization or stabilizing filaments effectively and would be related with the increase of contraction.
In conclusion, our present study show the gene expression profiling, based on a cDNA microarray analysis of normal and heat shock radial arteries, and suggest that the genes may play a pivotal role in the augmentation of vascular contraction after heat shock.
Figures and Tables
Fig. 1
Differentially expressed heat shock proteins. Total RNA was isolated from arterial tissues by using Trizol reagent. The expression levels of Hsp70, Hsp10, Crystallin=αB and Hsp60 mRNA were measured by real-time polymerase chain reaction after reverse transcription. The graphs show mean±SEM from pooled samples. *Statistically significant (*p<0.05) differences between control and heat-shock group. mRNA: messenger ribonucleic acid, HS: heat shock.
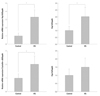
Acknowledgments
This work was supported by an Industry-Academy Cooperation research grant from the Korean Society of Circulation (2006).
References
1. Snoeckx LH, Cornelussen RN, Van Nieuwenhoven FA, Reneman RS, Van Der Vusse GJ. Heat shock proteins and cardiovascular pathophysiology. Physiol Rev. 2001. 81:1461–1497.
2. Park HW, Kown TG, Kim KY, Bae JH. Diabetes, insulin resistance and atherosclerosis surrogates in patients with coronary atherosclerosis. Korean Circ J. 2010. 40:62–67.
3. Lindquist S, Craig EA. The heat-shock proteins. Annu Rev Genet. 1988. 22:631–677.
4. Xu Q. Role of heat shock proteins in atherosclerosis. Arterioscler Thromb Vasc Biol. 2002. 22:1547–1559.
5. An HS, Bae EJ, Kim GB, et al. Pulmonary hypertension in preterm infants with bronchopulmonary dysplasia. Korean Circ J. 2010. 40:131–136.
6. Seo J, Kim M, Kim J. Identification of novel genes differentially expressed in PMA-induced HL-60 cells using cDNA microarrays. Mol Cells. 2000. 10:733–739.
7. Luo L, Salunga RC, Guo H, et al. Gene expression profiles of laser-captured adjacent neuronal subtypes. Nat Med. 1999. 5:117–122.
8. Eisen MB, Spellman PT, Brown PO, Botstein D. Cluster analysis and display of genome-wide expression patterns. Proc Natl Acad Sci U S A. 1998. 95:14863–14868.
9. Xu Q, Li DG, Holbrook NJ, Udelsman R. Acute hypertension induces heat-shock protein 70 gene expression in rat aorta. Circulation. 1995. 92:1223–1229.
10. Lee G, Oh Y, Lee J. Upregulation of heat shock proteins in the kidney in hypertension. Korean J Physiol Pharmacol. 2004. 8:147–151.
11. Meyer AS, Gillespie JR, Walther D, Millet IS, Doniach S, Frydman J. Closing the folding chamber of the eukaryotic chaperonin requires the transition state of ATP hydrolysis. Cell. 2003. 113:369–381.
12. Morton H. Early pregnancy factor: an extracellular chaperonin 10 homologue. Immunol Cell Biol. 1998. 76:483–496.
13. Cavanagh AC. Identification of early pregnancy factor as chaperonin 10: implications for understanding its role. Rev Reprod. 1996. 1:28–32.
14. Johnson GB, Brunn GJ, Platt JL. Activation of mammalian Toll-like receptors by endogenous agonists. Crit Rev Immunol. 2003. 23:15–44.
15. Wallin RP, Lundqvist A, Moré SH, von Bonin A, Kiessling R, Ljunggren HG. Heat-shock proteins as activators of the innate immune system. Trends Immunol. 2002. 23:130–135.
16. Kim IK, Park TG, Kim YH, Cho JW, Kang BS, Kim CY. Heat-shock response is associated with enhanced contractility of vascular smooth muscle in isolated rat aorta. Naunyn Schmiedebergs Arch Pharmacol. 2004. 369:402–407.
17. Groenen PJ, Merck KB, de Jong WW, Bloemendal H. Structure and modifications of the junior chaperone alpha-crystallin: from lens transparency to molecular pathology. Eur J Biochem. 1994. 225:1–19.
18. Datta SA, Rao CM. Differential temperature-dependent chaperone-like activity of alphaA- and alphaB-crystallin homoaggregates. J Biol Chem. 1999. 274:34773–34778.
19. Geeves MA, Fedorov R, Manstein DJ. Molecular mechanism of actomyosin-based motility. Cell Mol Life Sci. 2005. 62:1462–1477.
20. Barral JM, Hutagalung AH, Brinker A, Hartl FU, Epstein HF. Role of the myosin assembly protein UNC-45 as a molecular chaperone for myosin. Science. 2002. 295:669–671.
21. Srikakulam R, Winkelmann DA. Chaperone-mediated folding and assembly of myosin in striated muscle. J Cell Sci. 2004. 117(Pt 4):641–652.
22. Shirinsky VP, Vorotnikov AV, Birukov KG, et al. A kinase-related protein stabilizes unphosphorylated smooth muscle myosin minifilaments in the presence of ATP. J Biol Chem. 1993. 268:16578–16583.
23. Silver DL, Vorotnikov AV, Watterson DM, Shirinsky VP, Sellers JR. Sites of interaction between kinase-related protein and smooth muscle myosin. J Biol Chem. 1997. 272:25353–25359.
24. Lim KA, Kim KC, Cho MS, Lee BE, Kim HS, Hong YM. Gene expression of endothelin-1 and endothelin receptor A on monocrotaline-induced pulmonary hypertension in rats after bosentan treatment. Korean Circ J. 2010. 40:459–464.
25. Lees-Miller JP, Helfman DM. The molecular basis for tropomyosin isoform diversity. Bioessays. 1991. 13:429–437.
26. Gordon AM, Homsher E, Regnier M. Regulation of contraction in striated muscle. Physiol Rev. 2000. 80:853–924.
27. Cipolla MJ, Gokina NI, Osol G. Pressure-induced actin polymerization in vascular smooth muscle as a mechanism underlying myogenic behavior. FASEB J. 2002. 16:72–76.
28. Tang DD, Tan J. Downregulation of profilin with antisense oligodeoxynucleotides inhibits force development during stimulation of smooth muscle. Am J Physiol Heart Circ Physiol. 2003. 285:H1528–H1536.
29. Drees F, Pokutta S, Yamada S, Nelson WJ, Weis WI. Alpha-catenin is a molecular switch that binds E-cadherin-beta-catenin and regulates actin-filament assembly. Cell. 2005. 123:903–915.