Abstract
Purpose
To evaluate the usefulness of in vivo magnetic resonance (MR) imaging for tracking intravenously injected superparamagnetic iron oxide (SPIO)-labeled human umbilical vein endothelial cells (HUVECs) in an acute renal failure (ARF) rat model.
Materials and Methods
HUVECs were labeled with SPIO and poly-L-lysine (PLL) complex. Relaxation rates at 1.5-T MR, cell viability, and labeling stability were assessed. HUVECs were injected into the tail vein of ARF rats (labeled cells in 10 rats, unlabeled cells in 2 rats). Follow-up serial T2*-weighted gradient-echo MR imaging was performed at 1, 3, 5 and 7 days after injection, and the MR findings were compared with histologic findings.
Results
There was an average of 98.4±2.4% Prussian blue stain-positive cells after labeling with SPIO-PLL complex. Relaxation rates (R2*) of all cultured HUVECs at day 3 and 5 were not markedly decreased compared with that at day 1. The stability of SPIO in HUVECs was maintained during the proliferation of HUVECs in culture media. In the presence of left unilateral renal artery ischemia, T2*-weighted MR imaging performed 1 day after the intravenous injection of labeled HUVECs revealed a significant signal intensity (SI) loss exclusively in the left renal outer medulla regions, but not in the right kidney. The MR imaging findings at days 3, 5 and 7 after intravenous injection of HUVECs showed a SI loss in the outer medulla regions of the ischemically injured kidney, but the SI progressively recovered with time and the right kidney did not have a significant change in SI in the same period. Upon histologic analysis, the SI loss on MR images was correspondent to the presence of Prussian blue stained cells, primarily in the renal outer medulla.
Acute renal failure (ARF) remains a significant cause of morbidity and mortality in adults and children. Although developing a treatment for ARF has been a challenge, there have been some advances in preventative strategies and supportive measures (1). Cell therapy, including the use of stem cells and progenitor cells, has recently attracted a great deal of interest as a new therapeutic strategy for ARF (2). Recently, Brodsky et al. (3) demonstrated that implantation of human umbilical vein endothelial cells (HUVECs) resulted in functional protection from ischemic ARF. Important considerations for improving cell-based therapy for renal failure include the delivery of cells to the site of renal injury and successful engraftment. Noninvasive imaging techniques allow in vivo monitoring of cell location and can therefore be of great value for improving cell-based therapy for renal failure.
Evaluation of transplanted or injected therapeutic cells is currently conducted by histologic analysis.
Although histologic analysis allows direct visualization of therapeutic cells on tissue, it cannot be used for in vivo cell tracking. Advances in cell labeling techniques have allowed many researchers to track cells in vivo with molecular imaging such as bioluminescence, nuclear or MR imaging. Nowadays, there is an increasing interest in in vivo cell tracking with high-spatial-resolution magnetic resonance (MR) imaging due to its ability to depict detailed anatomy (4, 5). Superparamagnetic iron oxide (SPIO) particles, which have been used as a contrast agent in clinical medical imaging, are already being used to label cells for in vivo MR cell tracking (6-11). Different classes of transfection agents such as polycationic amines, dendrimers, and lipid-based agents have been employed to efficiently load cultured cells with enough SPIO for in vivo MR imaging (12). It has been reported that PLL, a polycationic amine, has little cytotoxicity and can be used for effective transfection efficiency in mammalian cells (12). The utility of MR cell tracking with SPIO-labeled stem cells has been shown in the experimental monitoring of cell-based therapies for myocardial infarction (9, 13), adult and neonatal mice brain,10 and a rat model of nephropathy (11). MR cell tracking imaging with inflammatory cells has also been applied to conditions such as graft rejection after transplantation (14) and adhesion of natural killer cells to tumors (15). To our knowledge, no study using MR imaging has been performed on tracking intravenously injected SPIO-labeled endothelial cells in rats with induced ARF, which may have a role in the recovery of deteriorated renal function.
Thus, the purpose of this study was to evaluate the usefulness of in vivo MR imaging for monitoring the distribution of HUVECs after intravenous injection in a rat model for unilateral ischemic acute renal failure.
Human umbilical vein endothelial cells (HUVECs) were prepared from human umbilical cords by collagenase digestion as previously described (16). The endothelial identity of the cultures was confirmed by the detection of factor VIII by immunofluoresence. HUVECs were maintained in M199 medium supplemented with 20% (vol/vol) fetal bovine serum at 37℃ in a 5% CO2 atmosphere. The primary cultured cells in this study were used between passages 2 and 4.
PLL hydrobromide (Sigma, St Louis, MO, USA) was used as the transfection agent. The PLL (1.5 mg/mL) was added to the culture media at a dilution of 1:1,000 and mixed with ferumoxides (Feridex 50 µg/mL; Wayne, NJ, USA) for 60 minutes at room temperature on a rotating shaker. These culture media containing the ferumoxides-PLL complex were added to the cells such that the final concentration of ferumoxides was 25 µg/mL and the final dilution of PLL was 1:2,000. The cell cultures were kept overnight at 37℃ in a 95% air per 5% CO2 atmosphere (17). After labeling, cells were washed two times and resuspended in complete media. Cell viability was maintained at greater than 95% in all instances and was monitored before and after labeling. There was no difference observed in cell viability (95%) between the ferumoxides-PLL labeled and unlabeled HUVECs.
Before injection, HUVECs were loaded with Green-fluorescent fluorescein diacetate (CellTracker, Molecular Probes, Eugene, OR) according to the manufacture's protocol. Briefly, confluent HUVECs were lifted with trypsin-EDTA and suspended. CellTracker (10 µM) was added to the HUVECs and HUVECS were incubated for 15 minutes at 37℃. After centrifugation at 1,500 rpm and 4℃, M199 media was added, and the HUVECs were incubated an additional 15 minutes at 37℃. After being washed two times with PBS, HUVECs were resuspended in PBS at a final concentration of 6×106 cells/mL. The cell suspension was kept on ice until transplantation, but for no longer than 30 minutes. Fluorescent images of the labeled cells were obtained to confirm the thoroughness of the cell labeling.
To study labeling stability, cultured HUVECs were divided into three groups, each with the same number of cells (1 × 106 cells each). HUVECs were labeled by means of incubation with ferumoxides-PLL complexes overnight and then were replated in a 10-cm culture dish. After 1, 3, and 5 days, all HUVECs were washed three times, treated with trypsin, and harvested. After centrifugation, HUVECs were then suspended in PBS for the MR relaxation measurements. All samples were placed in a head coil provided by the manufacturer and imaged with a clinical 1.5-T MR imager (Magnetom Symphony, Siemens Medical Systems, Erlangen, Germany). The T2* relaxation time was determined by using a multiple gradient-echo sequence, with a fixed repetition time of 1000 msec, flip angle of 30°, and 5 echoes starting at 7.5 msec. R2* (1/T2*) was deduced by fitting a single exponential function to the signal intensity versus TE data (TE of 7.5 - 60 msec and fixed TR). It has been well recognized that R2* depends linearly on the global iron concentration of the sample (18).
Inbred male Sprague-Dawley rats (Charles River Korea, Seoul, Korea) were maintained on standard laboratory chow and water ad libitum. ARF was induced by unilateral (left) renal artery ischemia followed by the reperfusion of blood flow. Briefly, rats (n=12) were anesthetized with an intramuscular injection of 100 mg/kg ketamine and 10 mg/kg xylazine. The animals were placed on a heating table to maintain constant body temperature. A midline incision was made and the left renal pedicle was exposed and clamped for 40 minutes. After the clamp was removed, the kidney was inspected for restoration of blood flow. After abdominal wall closure, HUVECs (6 × 106 cells) labeled with SPIO and CellTracker according to the above methods were diluted in 300-400 µL PBS and injected into the tail vein of the ARF rats. Rats (n=8) injected with labeled HUVECs were imaged at 1, 3, 5, and 7 days after injection with a 1.5-T MR imager. Rats (n=2) injected with unlabeled HUVECs and sham-operated rats (n=2) injected with labeled HUVECs were imaged 1 day after injection to evaluate signal intensity changes in the kidney. At days 1, 3, 5, and 7, the animals were sacrificed and both kidneys were harvested for histologic examination.
All in vivo MR images of the rats were obtained by a 1.5-T clinical MR imager (Magnetom Symphony, Siemens Medical Systems, Erlangen, Germany) using a small flexible coil with a width of 17 cm provided by the manufacturer. Rats were positioned prone in the flexible coil. Then, fat-suppressed, coronal, and transverse MR images were obtained using a gradient-echo sequence with 500/13 (TR/TE, msec), 30° flip angle, 75 mm field of view, 2.5 mm section thickness, 256 × 192 matrix, and eight signals were acquired to evaluate signal decay in the kidneys. Both kidneys were imaged on the same imaging plane. MR images of the rats were obtained after 1 × 106 HUVECs were injected through tail veins at days 1, 3, 5, and 7. After injection of labeled HUVECs, low signal intensity was observed predominantly in the outer medulla of the left kidney, in which ARF had been induced. Signal intensity values at the outer medulla and cortex of the left kidney were measured to calculate the ratio of signal decrease due to the presence of labeled HUVECs. Signal intensity values for low signal intensity regions were measured on serial MR images using the region of interest in the outer medulla of the left kidney. Also signal intensity values for high signal intensity were measured in the cortex of the left kidney. The ratio of signal decrease (Srsd) in the outer medulla of the left kidney was determined by using the equation Srsd = [(Sco - Som)/Sco] × 100, where Sco is the signal intensity value measured in the renal cortex and Som is the signal intensity value measured in the outer medulla of the kidney. The ratio of signal decrease in the right kidney was also measured with the same method as in the left kidney.
Renal tissue was prepared after in vivo MRI examination. The tissue blocks of rat kidney were fixed in 4% paraformaldehyde and processed for paraffin embedding. Histopathology was examined on 5 µm slides stained by hematoxylin and eosin, and Prussian blue staining for iron was used by implementing standard methods on the kidney sections from the ferumoxides-PLL labeled group (n = 8 with ARF, n = 2 sham operation) and unlabeled controls (n = 2). To examine Cell Tracker-labeled HUVECs, a fluorescent microscope (Carl Zeiss, Göttingen, Germany) was used on fixed frozen sections of kidney tissue.
Prussian blue stain for iron was performed on unlabeled and ferumoxides-PLL labeled HUVECs in culture (Fig. 1a). On average, there were 98.4±2.4% Prussian blue stain-positive cells after labeling with ferumoxides-PLL and 2.4±0.2% Prussian blue stain-positive cells without labeling with ferumoxides-PLL. With fluorescent microscopy, essentially all HUVECs labeled with CellTracker appeared green fluorescent, while unlabeled HUVECs were rarely green fluorescent (Fig. 1b). Electron microscopic examination showed the presence of SPIO nanoparticles in the endosomal vesicles of the HUVECs (Fig. 1c).
The labeling stability of HUVECs was maintained 5 days after ferumoxides-PLL labeling.
The relaxation rate (R2*) of SPIO-unlabeled HUVECs was 14.1 sec-1 and those of SPIO-labeled HUVECs at days 1, 3, and 5 were 25 sec-1, 21.3 sec-1 and 24.4 sec-1, respectively. The relaxation rate (R2*) of HUVECs at day 1 increased by over 50% in comparison with that of unlabeled HUVECs (the number of cells, 1 × 106). However, relaxation rates at days 3 and 5 were not markedly decreased compared with that at day 1 (Fig. 2). Stability of SPIO in HUVECs was maintained during proliferation of HUVECs in culture media.
After intravenous injection of labeled HUVECs, the presence of HUVECs in the kidney was detected by a regional signal intensity loss induced by the susceptibility effects of iron oxide particles. T2*-weighted MR images showed a signal intensity loss exclusively in the left renal outer medulla regions, but the right kidney did not show a significant change in signal intensity at day 1 (Fig. 3a). MR imaging at 3, 5, and 7 days after intravenous injection of HUVECs in rats with left unilateral renal artery ischemia initially showed a signal intensity loss in the left renal outer medulla regions, but then demonstrated a progressive recovery of signal intensity. The right kidney showed no significant change in signal intensity (Fig. 3b-d). Upon examination of serial MR images, the change in the ratio of signal intensity in the renal outer medulla HUVECs was in accordance with the recovery of the signal intensity loss observed on the serial T2*-weighted MR imaging (Fig. 4). The T2*-weighted MR images at day 1 showed no signal intensity loss in the medulla of both rat kidneys (n = 2) injected with unlabeled HUVECs and in the kidneys of sham-operated rats (n = 2) injected with labeled HUVECs.
One day after the operation and injection of labeled HUVECs in the rat, we removed the ischemically injured (left) kidneys. Sham-operated (right) kidneys were used as the control. Light microscopy showed that tubular dilatation, loss of brush border, and tubular cell loss were concentrated in the ischemically injured outer medulla of the left kidney, but the right control kidney was normal (Fig. 5a, b). Prussian blue stain-positive cells were rarely found in the cortex and medulla in the sham-operated kidney (Fig. 5c). However, Prussian blue stain-positive cells were found in the outer medulla of the injured kidney, where a signal intensity decrease was observed with MR imaging (Fig. 5d). We also performed a fluorescent microscopic examination to detect HUVECs labeled with CellTracker. In comparison to the right kidney (Fig. 5e), the left kidney had a significantly increased number of CellTracker-positive cells in the outer medulla (Fig. 5f).
We investigated the correlation between the histologic examination and MR imaging of the location of SPIO-labeled HUVECs. At 1 and 5 days after injection of SPIO-labeled HUVECs, we performed MR imaging and histologic examination of the left kidney. MR imaging at 1 day showed a signal intensity loss in the left renal outer medulla regions (Fig. 6a). Prussian blue stain-positive cells were found in the outer medulla of the left kidney (Fig. 6c). By fluorescent microscopy, CellTracker-positive HUVECs were found in the outer medulla of the left kidney (Fig. 6e). There was a strong correlation between the area of signal intensity loss and the location of Prussian blue stain-positive cells and CellTracker-positive HUVECs. MR imaging at 5 days after the injection of HUVECs showed a decreased signal intensity loss in the left renal outer medulla region (Fig. 6b). Prussian blue stain-positive cells and CellTracker-positive HUVECs were found in the same area at day 5 but were slightly decreased in number compared to those of day 1 (Fig. 6d, f).
Our results demonstrated that HUVECs can be successfully labeled in vitro using a commercially available SPIO and the transfection agent, PLL. Serial T2* MR imaging examination with a 1.5-T MR imager showed that the SPIO-labeled HUVECs could be detected 1 day after intravenous injection into an acute ischemically injured kidney. We also found that signal intensity loss due to the presence of magnetically labeled HUVECs could be followed up to 7 days with MR. The detection of HUVECs in injured kidneys on T2* MR images was confirmed by histologic and fluorescent microscopic examinations. Furthermore, in the right control kidney without acute renal ischemic injury, the signal loss due to labeled HUVECs was not observed on MR images and no cells were found in the kidneys by histologic examination.
MR imaging with SPIO particles, which have been used for the magnetic labeling of cells, has been shown to be a useful noninvasive method for detection of implanted or grafted cells (11). An SPIO particle usually consists of a polycrystalline magnetic core embedded within a polymer coating, such as dextran, carboxydextran, or polyethylene glycol of varying diameters (10-3500 nm) (19-21). SPIO agents with less than a 50 nm diameter have been used for molecular imaging applications in vivo (21). The present study demonstrates that ferumoxides-PLL complexes allow for the incorporation of SPIO into the endosomal vesicle of HUVECs. The results of in vitro labeling of HUVECs agree with those of Zhang et al, which showed the usefulness of iron oxide preparation for cell tracking (6). Zhang et al also reported that the toxicity of intracellular SPIO increased with higher doses of SPIO (≥ 200 µg/ml) in HUVECs because of the decrease in mitochondrial membrane potential. Cytotoxicity can be caused by the generation of oxygen free radicals, which are able to react with membrane lipids, initiating lipid peroxidation (6). In our study, we used a ferumoxide concentration of 50 µg/mL because higher doses of iron concentration may influence cell viability (18). We also demonstrated that the SPIO-labeled HUVECs did not show a significant relaxation rate change for 5 days, suggesting that the labeling stability of HUVECs can be maintained during serial MR examinations.
It is important to determine the optimal route for cell administration, which may be by intra-arterial or intravenous injection or by direct implantation. In our study, we used the intravenous route of cell administration, because it is easy and safe. Hauger el al. (11) reported that the intravenous route does not require arterial catheterization or contrast medium injection, which is advantageous for renal failure patients. However, the limitation of the intravenous route is that only a small proportion of cells can reach the target organ because injected cells may be trapped in the liver, lung or spleen. In contrast to the intravenous route, the intra-arterial route has the advantage of increasing the number of cells localizing to the target organ, which can prevent injected cells from hepatic sinusoidal trapping. But, more invasive intervention is usually required (11, 18). The number of SPIO-labeled cells in the target organ may determine the signal intensity loss of MR images. Therefore, intra-arterial injection of labeled cells can be advantageous for MR signal intensity changes because the number of cells delivered by intra-arterial injection is usually larger than that by intravenous injection. We injected 1 × 106 SPIO-labeled HUVECs intravenously to detect cells localizing to the kidney, and serial MR images showed a signal intensity loss in the acute ischemically injured kidney.
In acute renal ischemia, proximal tubular epithelial cell injury is a major pathophysiologic event (1). But, renal vascular changes including renal vascular endothelial injury and dysfunction may occur simultaneously after ischemic acute renal failure (22, 23). It has been demonstrated that tissue ischemia and cytokines mobilize bone marrow-derived endothelial progenitor cells (24) and that circulating endothelial cells of hematopoietic origin are recruited to the ischemic areas to form adult blood vessels (25). Interestingly, our results show that labeled HUVECs were found only in the ischemically injured left kidney, but not in the sham-operated right kidney. Our results suggest that unilateral ischemic injury significantly stimulates the recruitment of transplanted endothelial cells to the injured kidney.
Although we did not analyze the functional changes after ARF, we used in vivo cell tracking with SPIO particles and a commercially available 1.5-T MR imaging system to show that HUVECs may be engrafted in the outer medulla of an injured kidney for systemic treatment of renal failure. To our knowledge, this is the first report of systemic intravenous cell grafting and in vivo MR imaging of SPIO-labeled HUVECs within an ischemically injured kidney.
There were several limitations in this study. We demonstrated only the localization of SPIO-labeled HUVECs in an acute ischemically injured kidney with MR imaging. Uncovering the mechanism by which HUVECs localize to the ischemically injured kidney was beyond the scope of this study. Also, we followed the presence of SPIO-labeled HUVECs for up to 7 days, but the recovery period for acute ischemic renal failure may exceed 7 days. Further, we did not analyze the relationship between HUVEC recruitment in the injured kidney and renal function improvement.
MR imaging appears to be useful for in vivo monitoring of intravenously injected SPIO-labeled HUVECs in an ischemically injured rat kidney, which has been used as a model of cell therapy.
Figures and Tables
Fig. 1
Light, fluorescent and electron microscopic findings for labeled and unlabeled HUVECs.
a. Light micrograph of Prussian blue-stained HUVECs. Unlabeled cells (upper panel) show no iron staining after a Prussian blue stain and cells labeled with ferumoxides-PLL complex (lower panel) show blue/iron staining (arrows) in the cytoplasm. Bar = 50 µm.
b. Fluorescent micrograph of HUVECs unlabeled (upper panel) and labeled (lower panel) with CellTracker. HUVECs were incubated with Celltracker for 15 minutes. Essentially all HUVECs labeled with CellTracker appear green fluorescent. Bar = 50 µm.
c. Electron micrograph of SPIO labeled HUVECs showed electron dense SPIO particles in the cytoplasmic endosomal vesicles (×12,000).
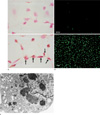
Fig. 2
Labeling stability. Relaxation rates (R2*) of all HUVECs (the number of cells, 1 × 106 cells) after labeling with SPIO at days 1, 3, and 5 were similar to each other. However, the relaxation rate (R2*) of HUVECs at day 1 was increased by over 50% in comparison with that of unlabeled HUVECs (the number of cells, 1 × 106).
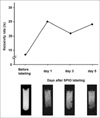
Fig. 3
Coronal T2*-weighted MR images of rat kidney.
a-d. Serial images obtained after 1, 3, 5, and 7 days, respectively. a. Finding at the T2*-weighted postinjection MR image showed a signal intensity loss exclusively in the left renal outer medulla regions (arrow), but the right kidney did not show a significant change in signal intensity at 1 day in a kidney with unilateral renal artery ischemia. b-d. MR images at 3, 5, and 7 days after intravenous injection of HUVECs into rats with unilateral renal artery ischemia showed a signal intensity loss in the left renal outer medulla regions that progressively faded (arrow), and the right kidney showed no significant change of signal intensity.
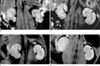
Fig. 4
Graph of serial T2*-ROI measurements of the renal cortex and medulla after HUVECs injection. After injection of the labeled cells, an increase in the T2* ROI was observed. Serial T2*-ROI was gradually decreased at 3, 5, and 7 days after SPIO-labeled HUVECs injection.
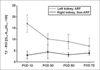
Fig. 5
Light and fluorescent microscopic findings 1 day after unilateral renal artery ischemia.
a, b. Light microscopic examination showed that the control right kidney was normal (a) but, tubular dilatation, loss of brush border and tubular cell loss were concentrated in the outer medulla of the left kidney (b).
c, d. Light micrograph of Prussian blue stain shows that blue colored cells were found at the outer medulla of the left kidney 1 day after unilateral renal artery ischemia (d) but not in the right kidney (c).
e, f. Fluorescent microscopy showed that CellTracker-positive HUVECs were found in the outer medulla of left kidney (f) but not in the right kidney (e). (H & E stain, Bar = 50 µm.)
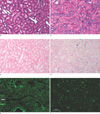
Fig. 6
MR images and light and fluorescent microscopic findings of an ischemically injured kidney after injection of SPIO-labeled HUVECs at days 1 and 5. MR imaging examination on day 1 showed a signal intensity loss in the left renal outer medulla regions (a). Prussian blue stain-positive cells were found at the outer medulla of the left kidney (c). By fluorescent microscopic examination, the CellTracker-positive HUVECs were found in the outer medulla of the left kidney (e). There was a strong correlation between signal intensity loss area and the location of Prussian blue stain-positive cells and CellTracker-positive HUVECs. MR imaging 5 days after injection of HUVECs showed a decreased signal intensity loss in the left renal outer medulla region (b). Prussian blue stain-positive cells and CellTracker-positive HUVECs were found in the same area, but slightly decreased in numbers compared to day 1 (d and f).
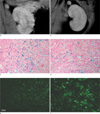
References
1. Thadhani R, Pascual M, Bonventre JV. Acute renal failure. N Engl J Med. 1996; 334:1448–1460.
2. Rookmaaker MB, Verhaar MC, van Zonneveld AJ, Rabelink TJ. Progenitor cells in the kidney: biology and therapeutic perspectives. Kidney Int. 2004; 66:518–522.
3. Brodsky SV, Yamamoto T, Tada T, et al. Endothelial dysfunction in ischemic acute renal failure: rescue by transplanted endothelial cells. Am J Physiol Renal Physiol. 2002; 282:F1140–F1149.
4. Sun R, Dittrich J, Le-Huu M, et al. Physical and biological characterization of superparamagnetic iron oxide- and ultrasmall superparamagnetic iron oxide-labeled cells: a comparison. Invest Radiol. 2005; 40:504–513.
5. Frank JA, Anderson SA, Kalsih H, et al. Methods for magnetically labeling stem and other cells for detection by in vivo magnetic resonance imaging. Cytotherapy. 2004; 6:621–625.
6. Zhang Z, van den Bos EJ, Wielopolsk PA, et al. In vitro imaging of single living human umbilical vein endothelial cells with a clinical 3.0-T MRI scanner. Magma. 2005; 18:175–185.
7. Bulte JW, Zhang S, van Gelderen P, et al. Neurotransplantation of magnetically labeled oligodendrocyte progenitors: magnetic resonance tracking of cell migration and myelination. Proc Natl Acad Sci USA. 1999; 96:15256–15261.
8. Bulte JW, Arbab AS, Douglas T, Frank JA. Preparation of magnetically labeled cells for cell tracking by magnetic resonance imaging. Methods Enzymol. 2004; 386:275–299.
9. Kraitchman DL, Heldman AW, Atalar E, et al. In vivo magnetic resonance imaging of mesenchymal stem cells in myocardial infarction. Circulation. 2003; 107:2290–2293.
10. Magnitsky S, Watson DJ, Walton RM, et al. In vivo and ex vivo MRI detection of localized and disseminated neural stem cell grafts in the mouse brain. Neuroimage. 2005; 26:744–754.
11. Hauger O, Frost EE, van Heeswijk R, et al. MR evaluation of the glomerular homing of magnetically labeled mesenchymal stem cells in a rat model of nephropathy. Radiology. 2006; 238:200–210.
12. Arbab AS, Yocum GT, Wilson LB, et al. Comparison of transfection agents in forming complexes with ferumoxides, cell labeling efficiency, and cellular viability. Mol Imaging. 2004; 3:24–32.
13. Himes N, Min JY, Lee R, et al. In vivo MRI of embryonic stem cells in a mouse model of myocardial infarction. Magn Reson Med. 2004; 52:1214–1219.
14. Ho C, Hitchens TK. A non-invasive approach to detecting organ rejection by MRI: monitoring the accumulation of immune cells at the transplanted organ. Curr Pharm Biotechnol. 2004; 5:551–566.
15. Kircher MF, Allport JR, Graves EE, et al. In vivo high resolution three-dimensional imaging of antigen-specific cytotoxic T-lymphocyte trafficking to tumors. Cancer Res. 2003; 63:6838–6846.
16. Sung MJ, Kim W, Ahn SY, et al. Protective effect of alpha-lipoic acid in lipopolysaccharide-induced endothelial fractalkine expression. Circ Res. 2005; 97:880–890.
17. Arbab AS, Bashaw LA, Miller BR, et al. Characterization of biophysical and metabolic properties of cells labeled with superparamagnetic iron oxide nanoparticles and transfection agent for cellular MR imaging. Radiology. 2003; 229:838–846.
18. Bos C, Delmas Y, Desmoulière A, et al. In vivo MR imaging of intravascularly injected magnetically labeled mesenchymal stem cells in rat kidney and liver. Radiology. 2004; 233:781–789.
19. Jung CW, Jacobs P. Physical and chemical properties of superparamagnetic iron oxide MR contrast agents: ferumoxides, ferumoxtran, ferumoxsil. Magn Reson Imaging. 1995; 13:661–674.
20. Artemov D. Molecular magnetic resonance imaging with targeted contrast agents. J Cell Biochem. 2003; 90:518–524.
21. Thorek DL, Chen AK, Czupryna J, Tsourkas A. Superparamagnetic iron oxide nanoparticle probes for molecular imaging. Ann Biomed Eng. 2006; 34:23–38.
22. Basile DP, Donohoe D, Roethe K, Osborn JL. Renal ischemic injury results in permanent damage to peritubular capillaries and influences long-term function. Am J Physiol Renal Physiol. 2001; 281:F887–F899.
23. Sutton TA, Fisher CJ, Molitoris BA. Microvascular endothelial injury and dysfunction during ischemic acute renal failure. Kidney Int. 2002; 62:1539–1549.
24. Takahashi T, Kalka C, Masuda H, et al. Ischemia- and cytokine-induced mobilization of bone marrow-derived endothelial progenitor cells for neovascularization. Nat Med. 1999; 5:434–438.
25. Crosby JR, Kaminski WE, Schatteman G, et al. Endothelial cells of hematopoietic origin make a significant contribution to adult blood vessel formation. Circ Res. 2000; 87:728–730.