Abstract
Purpose
Erectile dysfunction (ED) remains a major complication from cavernous nerve injury during radical prostatectomy. Recently, stem cell treatment for ED has been widely reported. This study was conducted to investigate the availability, differentiation into functional cells, and potential of human muscle-derived stem cells (hMDSCs) and human adipose-derived stem cells (hADSCs) for ED treatment.
Materials and Methods
We compared the neural differentiation of hMDSCs and hADSCs. Human muscle and adipose tissues were digested with collagenase, followed by filtering and centrifugation. For neural induction, isolated hMDSCs and hADSCs were incubated in neurobasal media containing forskolin, laminin, basic-fibroblast growth factor, and epidermal growth factor for 5 days. Following neural induction, hMDSCs and hADSCs were differentiated into neural cells, including neurons and glia, in vitro.
Results
In neural differentiated hMDSCs (d-hMDSCs) and differentiated hADSCs (d-hADSCs), neural stem cell marker (nestin) showed a significant decrease by immunocytochemistry, and neuronal marker (β-tubulin III) and glial marker (GFAP) showed a significant increase, compared with primary hMDSCs and hADSCs. Real-time chain reaction analysis and Western blotting demonstrated significantly elevated levels of mRNA and protein of β-tubulin III and GFAP in d-hADSCs compared with d-hMDSCs.
Erectile dysfunction (ED) remains a common cause of significant postoperative morbidity in patients undergoing radical therapies for prostate cancers or other pelvic malignancies, because the cavernous nerve can be inadvertently axotomized, lacerated, or stretched at the time of surgery. Although techniques such as nerve-sparing radical prostatectomy, nerve grafting, and robotic-assisted radical prostatectomy have been developed in which the cavernous nerve is largely preserved, postoperatively, patients still face a high incidence of ED. In recent years, stem cell therapy has evolved as a potential therapy in the prevention of ED following cavernous nerve injury. The multilineage differentiation potential of stem cells can contribute to regenerate the damaged cavernous nerve, thereby providing an autologous and curative therapeutic option [1].
Although bone marrow cells have the ability to differentiate into neurons, limitations hinder the widespread application of these cells [2,3]. Among the various stem cells, human muscle-derived stem cells (hMDSCs) and human-adipose tissue derived stem cells (hADSCs) have great potential in stem cell therapy because of their abundance, ease of isolation, and low immunogenicity [4]. In addition, hMDSCs and hADSCs have a considerable capacity for self-renewal and can differentiate into neural lineages [5-9]. Although many studies on the neurogenic trans-differentiation of hMDSCs and hADSCs have been conducted, no in vitro studies are available for comparison of the neurogenic capacity of these cells. In this study, therefore, we compared the neuronal differentiation capacity of hMDSCs with that of hADSCs in vitro.
Human adipose tissues were obtained by simple liposuction from the abdominal subcutaneous fat of donors. Subcutaneous adipose tissues were washed with phosphate- buffered saline (PBS) and digested with 1 mg/ml collagenase under gentle agitation for 60 minutes at 37℃. Next, the digested tissues were filtered through a 100 µm nylon mesh in order to remove cellular debris and centrifuged at 1,500 rpm for 5 min to obtain a pellet. The pellet was re-suspended in RCME (RNL Bio, Seoul, Korea) or DMEM containing 10% fetal bovine serum (FBS). The cell suspension was re-centrifuged at 1,500 rpm for 5 minutes. The supernatant was discarded and the cell pellet was collected. The cell fraction was cultured overnight at 37℃ in 5% CO2 in RCME or DMEM. Adhesion of cells was checked under a microscope the next day. The procedure for preparation of hADSCs was performed under GMP conditions in the Stem Cell Research Center of RNL Bio.
Using scalpels, hMDSCs isolated from the rectus muscles of humans were removed and minced into a coarse slurry. Muscle tissues were enzymatically dissociated in collagenase type XI (Sigma, St. Louis, MO, USA), dispase (Invitrogen, Carlsbad, CA, USA), and trypsin-EDTA (Invitrogen). After physical and enzymatic dissociations, muscle-derived cells were centrifuged and re-suspended in proliferation medium (containing DMEM [Gibco, Grand Island, NY, USA], 10% horse serum, 10% FBS, 1% chick embryo extract, and 1% penicillin-streptomycin). For isolation of SMNPs, cells were plated in collagen-coated flasks (PP1). After 2 hours, cells non-adherent in PP1 were transferred into the next flasks (PP2) and incubated for 16 hours. No adherent cells were observed in PP3; therefore, the serial transfer of non-adherent cells was terminated at this point. During cultivation, two morphologically different cell populations were identified. These were fibroblast-like cells and round-shaped cells.
PP1 flasks initially contained a high proportion of fibroblast- like cells. PP2 and PP3 flasks contained approximately 30%, 10%, and <1% of fibroblast-like cells, respectively. Further purification of weakly adherent cells (primarily round-shaped cells) was performed on cells adherent in PP2 and PP3 flasks. Cells were trypsinized for 3 min, after which suspended cells from PP2 and PP3 flasks were combined and re-plated in a fresh flask. As these cells proliferated in proliferation medium for 3 to 4 days, the cells formed additional colonies.
Both hMDSCs and hADSCs (1x105cells) were grown as adherent cultures in DMEM supplemented with 10% FBS and 1% penicillin-streptomycin at 37℃ in 5% CO2. After 24 hours, cells were washed with PBS and plated in neurobasal media, 1% penicillin, and 50 µM/ml of forskolin and 1 µg/ml laminin, 20 ng/ml basic fibroblast growth factor (bFGF), and 20 ng/ml epidermal growth factor (EGF) with 2% FBS.
Both hMDSCs and hADSCs were placed and grown on poly-L-lysine-coated cover slips for 5 days. After 5 days, these cells were washed with PBS, fixed with 4% paraformaldehyde for 10 minutes at room temperature, washed with PBS again, and then permeabilized with 0.1% Triton-X 100. After washing twice with PBS, cells were blocked by incubation with 10% normal goat serum for 1 hour at room temperature. Nestin (1:100; Abcam, Combride, UK), β-tubulin III (Tuj1, 1:500; Abcam, Combride, UK), and GFAP (1:100; Abcam, Combride, UK) were then added. Primary antibodies were incubated overnight at 4℃. After washing, the cells were treated with Alexa 488-conjugated goat anti-mouse antibody as a secondary antibody, followed by incubation at room temperature for 1 hour. Nuclei were stained with DAPI for cell counting, and the cells were observed under a fluorescence microscope.
RNA was extracted from hMDSCs, differentiated hMDSCs (d-hMDSCs), hADSCs, and differentiated hADSCs (dhADSCs). Total RNA was prepared by using Trizol according to the manufacturer's protocol. First-strand cDNA was synthesized by using Oligo dT primer and Superscript II reverse transcriptase according to the manufacturer's instructions. Real-time polymerase chain reaction analysis (PCR) was performed by using RT SYBR Green/ROX qPCR Master Mix using the Real-time Thermal Cycler (50℃/2 min, 95℃/10 min, [95℃/15 s, 60℃/30 s, 72℃/30 s - 40 cycles], 95℃/15 s, 60℃/1 min, 95℃/15 s).
Protein was homogenized in ice-cold lysis buffer containing 20 mM Tris-Cl, pH 8.0, 150 mM NaCl, 1 mM EDTA, 1% Triton X-100, 10 M leupeptin, 20 g/ml chymostatin, and 2 mM PMSF. Following centrifugation at 12,000 xg for 30 minutes at 4℃, the supernatant was extracted and quantified by use of the bicinchoninic acid (BCA) protein assay kit (Pierce, Rockford, IL, USA) and then electrophoresed on an SDS-PAGE gel. After transfer to a nitrocellulose membrane, the membrane was blocked with 5% skim milk and then incubated with antibody against nestin (1:1,000; Abcam), β-tubulin III (1:1,000; Abcam), GFAP (1:1,000;Abcam), or β-actin, followed by incubation with the corresponding secondary antibody conjugated to horseradish peroxidase. The ECL method (Amersham, Arlington Heights, IL, USA) was used for development of protein bands.
hMDSCs and hADSCs were round in monolayer cultures before neural induction (Fig. 1a and i). In immunohistochemical staining, hMDSCs and hMDSCs were strongly positive for neural stem cell marker (nestin), but weakly positive for neuronal marker (β-tubulin III) and glial marker (GFAP) (Fig. 1b-d and j-l).
Following 5 days of neural induction, hMDSCs and hADSCs displayed a differentiated phenotype in morphology (Fig. 1e and n). Immunohistochemistry showed that nestin expression was decreased, and β-tubulin III and GFAP expression were increased (Fig. 1f-h and m-p). There was no significant difference in nestin expression between d-hMDSCs and d-hADSCs; however, β-tubulin III and GFAP expression in d-hADSCs was increased compared with that in d-hMDSCs. According to cell morphology and staining, hADSCs had a higher phenotype and neurogenic potential than did hMDSCs.
To dissect the neurogenic potential of hMDSCs and hADSCs at the molecular level, the mRNA levels of nestin, β-tubulin III, and GFAP were detected by real-time PCR, normalized to the housekeeping GAPDH. Nestin mRNA levels in d-hMDSCs and d-hADSCs were approximately 50% lower than the expression in hMDSCs and hADSCs, respectively. There was no significant difference in nestin mRNA expression between d-hMDSCs and d-hADSCs (Fig. 2A). However, β-tubulin III and GFAP mRNA expression in d-hADSCs was significantly higher than that in d-hMDSCs (Fig. 2B and 2C).
We also investigated the protein expression of nestin, β-tubulin III, and GFAP in differentiated hMDSCs and hADSCs by Western blot analysis (Fig. 3A). The protein expression of nestin, β-tubulin III, and GFAP before and after differentiation showed almost the same tendency with mRNA expression. Protein expression of nestin in the hMDSCs and hADSCs was significantly decreased after neuronal induction (Fig. 3B). β-tubulin III and GFAP protein expression in d-hADSCs was significantly higher than that in d-hMDSCs (Fig. 3C and 3D).
This study compared the neurogenic potential of hMDSCs and hADSCs by detecting several neuron cell markers. Our data suggest that differentiated hADSCs have higher neuronal/glial marker expression than do hMDSCs and thereby are more suitable for nerve regeneration.
The incidence of ED after radical prostatectomy by cavernous nerve injury remains high. Recently, stem cell treatment has been regarded as a potential treatment for ED [1,4]. In recent years, tissue engineering strategies have combined living cells and scaffold materials for the development of biological substitutes that can restore tissue functions. Both natural and synthetic materials have been fabricated for transplantation of stem cells and their specific differentiation into muscles, bones, cartilage, and neuron-like cells. Studies have shown that hMDSCs and hADSCs can be induced into adipocytes, osteoblasts, chondrocytes, myocytes, hepatocytes, endotheliocytes [5-9], and neuron-like cells [2,10-13]. The surface phenotype of hMDSCs and hADSCs is similar to that of bone marrow-derived stromal cells [2,3]. Previous reports have indicated that neural differentiation of hMDSCs and hADSCs is achieved with different experimental protocols, including the use of chemical agents, such as forskolin [11,14-17], laminin [11,14,18], EGF, and bFGF [11,14,17,19]. bFGF is known to generate neural precursor cells with a greater capacity for neuronal differentiation. EGF and neurotrophic factor are reported to restrict astrocyte lineages. Forskolin is a commonly used agent to increase the intracellular levels of cyclic adenosine monophosphate (cAMP) by activating the enzyme adenylyl cyclase. Laminin-stimulated levels of tyrosine hydroxylase of chromaffin cells were associated with their conversion to adrenergic sympathetic cells. Laminin has differential action in the proliferation, survival, and differentiation of cultured neuronal cells. Although forskolin, laminin, EGF, and bFGF enhanced neuron-like cell production, the results were greatly improved when the combination was added to the medium.
We succeeded in inducing the neural differentiation of hMDSCs and hADSCs by treatment with forskolin, laminin, b-FGF, and EGF. In the present study, the neural differentiation of hMDSCs and hADSCs was observed for several days. The neuronal phenotype at 5 days following neural induction was observed by staining for nestin-negative and β-tubulin III- and GFAP-positive. We found that d-hMDSCs and d-hADSCs displayed similar immunocytochemical changes following neural induction. hMDSCs and hADSCs grown under control conditions expressed low β-tubulin III and GFAP but detectable levels of nestin. After 5 days of neural induction, we detected strong staining for β-tubulin III as well as GFAP in d-hADSCs. Real-time PCR and Western blotting confirmed that the number of undifferentiated hMDSCs and hADSCs expressing cell type-specific markers was very low. Marker-positive cells showed a considerable increase with neural supplements. Neurons were observed in cultures when compared with that of nestin-negative and β-tubulin III-, GFAP-positive. Our results showed that differentiated muscle- or adipose-derived stem cells have morphological and phenotypic characteristics that are similar to those of neural cells. In this study, we confirmed that the ability of hADSCs to differentiate into neural cells was better than that of hMDSCs.
In summary, we have shown that both hMDSCs and hADSCs have morphological and phenotypic characteristics similar to those of neural cells. hADSCs have more neurogenic differentiation potential than do hMDSCs. Therefore, hADSCs seem to be an ideal alternative source of stem cells for cavernous nerve regeneration.
Figures and Tables
FIG. 1
Morphology and immunocytochemistry of nestin, β-tubulin III, and GFAP before and after human muscle-derived stem cells (hMDSCs) and human-adipose tissue derived stem cells (hADSCs) differentiation. Morphology and double fluorescent immunocytochemistry for nestin (green), β-tubulin III (green), and GFAP (green) shown in (A) hMDSCs, differentiated hMDSCs (d-hMDSCs), and (B) hADSCs, differentiated hADSCs (d-hADSCs). Nuclei are stained with DAPI (blue). Magnification is ×100.
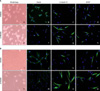
FIG. 2
mRNA expression of nestin, β-tubulin III, and GFAP before and after human muscle-derived stem cells (hMDSCs) and human-adipose tissue derived stem cells (hADSCs) differentiation. (A) Nestin, (B) β-tubulin III, and (C) GFAP mRNA levels in hMDSCs, differentiated hMDSCs (dhMDSCs), hADSCs, and differentiated hADSCs (d-hADSCs) were quantified by real-time chain reaction analysis analysis. GAPDH was used as the internal control gene. Data are the mean±SD of three different cultures of stem cells (ap<0.01).
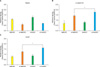
FIG. 3
Protein expression of nestin, β-tubulin III, and GFAP before and after human muscle-derived stem cells (hMDSCs) and human-adipose tissue derived stem cells (hADSCs) differentiation. (A) Nestin, β-tubulin III, and GFAP protein expression in hMDSCs, differentiated hMDSCs (d-hMDSCs), hADSCs, and differentiated hADSCs (d-hADSCs) were determined by Western blot analysis. Blots were probed with β-actin to show equal loading. The bar graphs show the relative levels of (B) nestin, (C) β-tubulin, and (D) GFAP protein in hMDSCs, d-hMDSCs, hADSCs, and d-hADSCs normalized to β-actin (n=3). Mean±SD, ap<0.01.
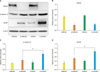
Notes
References
1. Lin G, Banie L, Ning H, Bella AJ, Lin CS, Lue TF. Potential of adipose-derived stem cells for treatment of erectile dysfunction. J Sex Med. 2009. 6:Suppl 3. 320–327.
2. Zurita M, Vaquero J, Oya S, Bonilla C, Aguayo C. Neurotrophic schwann-cell factors induce neural differentiation of bone marrow stromal cells. Neuroreport. 2007. 18:1713–1717.
3. Zurita M, Bonilla C, Otero L, Aguayo C, Vaquero J. Neural transdifferentiation of bone marrow stromal cells obtained by chemical agents is a short-time reversible phenomenon. Neurosci Res. 2008. 60:275–280.
4. Nolazco G, Kovanecz I, Vernet D, Gelfand RA, Tsao J, Ferrini MG, et al. Effect of muscle-derived stem cells on the restoration of corpora cavernosa smooth muscle and erectile function in the aged rat. BJU Int. 2008. 101:1156–1164.
5. Strem BM, Hicok KC, Zhu M, Wulur I, Alfonso Z, Schreiber RE, et al. Multipotential differentiation of adipose tissue-derived stem cells. Keio J Med. 2005. 54:132–141.
6. Krampera M, Marconi S, Pasini A, Galiè M, Rigotti G, Mosna F, et al. Induction of neural-like differentiation in human mesenchymal stem cells derived from bone marrow, fat, spleen and thymus. Bone. 2007. 40:382–390.
7. Alessandri G, Pagano S, Bez A, Benetti A, Pozzi S, Iannolo G, et al. Isolation and culture of human muscle-derived stem cells able to differentiate into myogenic and neurogenic cell lineages. Lancet. 2004. 364:1872–1883.
8. Jiang Y, Vaessen B, Lenvik T, Blackstad M, Reyes M, Verfaillie CM. Multipotent progenitor cells can be isolated from postnatal murine bone marrow, muscle, and brain. Exp Hematol. 2002. 30:896–904.
9. Casteilla L, Dani C. Adipose tissue derived cells: from physiology to regenerative medicine. Diabetes Metab. 2006. 32:393–401.
10. Safford KM, Hicok KC, Safford SD, Halvorsen YD, Wilkison WO, Gimble JM, et al. Neurogenic differentiation of murine and human adipose-derived stromal cells. Biochem Biophys Res Commun. 2002. 294:371–379.
11. Schultz SS, Lucas PA. Human stem cells isolated from adult skeletal muscle differentiate into neural phenotypes. J Neurosci Methods. 2006. 152:144–155.
12. Jiang L, Zhu JK, Liu XL, Xiang P, Hu J, Yu WH. Differentiation of rat adipose tissue derived stem cells into Schwann-like cells in vitro. Neuroreport. 2008. 19:1015–1019.
13. Zavan B, Michelotto L, Lancerotto L, Della Puppa A, D'Avella D, Abatangelo G, et al. Neural potential of a stem cell population in the adipose and cutaneous tissues. Neurol Res. 2010. 32:47–54.
14. Vourc'h P, Romero-Ramos M, Chivatakarn O, Young HE, Lucas PA, El-Kalay M, et al. Isolation and characterization of cells with neurogenic potential from adult skeletal muscle. Biochem Biophys Res Commun. 2004. 317:893–901.
15. Kingham PJ, Kalbermatten DF, Mahay D, Armstrong SJ, Wiberg M, Terenghi G. Adipose-derived stem cells differentiate into a schwann cell phenotype and promote neurite outgrowth in vitro. Exp Neurol. 2007. 207:267–274.
16. Safford KM, Safford SD, Gimble JM, Shetty AK, Rice HE. Characterization of neuronal/glial differentiation of murine adipose-derived adult stromal cells. Exp Neurol. 2004. 187:319–328.
17. Xu Y, Liu L, Li Y, Zhou C, Xiong F, Liu Z, et al. Myelin-forming ability of schwann cell-like cells induced from rat adipose-derived stem cells in vitro. Brain Res. 2008. 1239:49–55.
18. Romero-Ramos M, Vourc'h P, Young HE, Lucas PA, Wu Y, Chivatakarn O, et al. Neuronal differentiation of stem cells isolated from adult muscle. J Neurosci Res. 2002. 69:894–907.
19. Xu Y, Liu Z, Liu L, Zhao C, Xiong F, Zhou C, et al. Neurospheres from rat adipose-derived stem cells could be induced into functional Schwann cell-like cells in vitro. BMC Neurosci. 2008. 9:21.