Abstract
Prostate cancer (PCa) is the most commonly diagnosed visceral cancer in men and is responsible for the second highest cancer-related male mortality rate in Western countries, with increasing rates being reported in Korea, Japan, and China. Considering the low sensitivity of prostate-specific antigen (PSA) testing, it is widely agreed that reliable, age-independent markers of the presence, nature, and progression of PCa are required to facilitate diagnosis and timely treatment. Metabolomics or metabonomics has recently emerged as a novel method of PCa detection owing to its ability to monitor changes in the metabolic signature, within biofluids or tissue, that reflect changes in phenotype and function. This review outlines the physiology of prostate tissue and prostatic fluid in health and in malignancy in relation to metabolomics as well as the principles underlying the methods of metabolomic quantification. Promising metabolites, metabolic profiles, and their correlation with the presence and stage of PCa are summarized. Application of metabolomics to biofluids and in vivo quantification as well as the direction of current research in supplementing and improving current methods of detection are discussed. The current debate in the urology literature on sarcosine as a potential biomarker for PCa is reviewed and discussed. Metabolomics promises to be a valuable tool in the early detection of PCa that may enable earlier treatment and improved clinical outcomes.
Prostate cancer (PCa) is the most commonly diagnosed visceral cancer in men and is responsible for the second highest cancer-related male mortality rate in Western countries, with increasing rates being reported in Korea, Japan, and China [1-4]. Such high rates of mortality are attributed to the nature of the disease and its range in distribution and varied levels of aggressive behavior [5]. Current treatment focuses on early detection of PCa to enable early intervention which in turn enhances the likelihood of treatment success [6].
Currently, serum prostate-specific antigen (PSA) remains the standard initial test for PCa detection, although controversy surrounds its limited sensitivity and specificity [7-12]. Moreover, PSA does not provide prognostic information [10,13]. The strong positive association of PSA with age [14] and other prostatic conditions, including benign prostatic hyperplasia (BPH) [15,16], inevitably leads to many false-positive results. False-negative results are also common, as no reliable cutoff value exists for PSA to rule out the presence of PCa [9]. In addition, the current method of definitive diagnosis, biopsy, misses many tumors [17]. In this context, it is widely agreed that reliable, age-independent markers of the presence of PCa, its nature, and its progression beyond the prostate are required to enable individual treatment to occur in a timely manner [18-21]. Metabolomics has recently emerged as a promising method of PCa detection [22], one that may supplement, or even eventually replace, current PSA testing in providing diagnostic accuracy.
The objective of this article was to review the current status of metabolomics in facilitating the early diagnosis of PCa, in addition to other benefits it may provide for clinicians in the future.
Metabolomics can detect and quantify low-molecular-weight metabolites produced by living cells [5]. Metabolomics has been described as "the quantitative measurement of the dynamic multiparametric metabolic response of living systems to pathophysiological stimuli or genetic modification" [23]. The metabolites of living cells are seen as the end products of the biological hierarchy starting with activated genes (genome) and extending over the collection of gene transcripts (transcriptome) and proteins (proteome). This process is summarized in Fig. 1.
Neoplastic transformation is considered to necessitate metabolic alterations to provide the bioenergetic and synthetic requirements of malignancy [24]. It is on this basis that alterations to the metabolic signatures within biofluids or tissues reflect changes in phenotype and function and are key to differentiating tumors from normal tissues [25-27]. Moreover, it is believed that metabolic alterations precede neoplastic proliferation. Thus, if such precancerous metabolic alterations are detectable, early intervention may even prevent cancer development or minimize neoplastic proliferation and invasion of local or distant structures. Metabolomics has enormous potential as a less-invasive screening agent via chemical analysis of biofluids and other biological samples, such as biopsies or tissue samples, or through molecular imaging techniques such as magnetic resonance imaging (MRI) and positron emission tomography (PET) [28].
In the prostate, the peripheral zone (PZ) constitutes not only the majority of the volume (70%) and function, but also the majority of malignancy rates (85%) [29]. The function of the PZ epithelium is the production, storage, and secretion of prostatic fluid, which is composed of citrate, polyamines (spermine, myoinositol), and PSA [30-32]. The citrate concentration in the PZ is 20-70 times that in blood plasma, whereas citrate levels in prostatic fluid are 400-1,500 higher than in blood plasma [29]. Production of these metabolites makes the PZ epithelium unique among human cells, because intracellular metabolism significantly favors citrate synthesis over citrate utilization. Normally in cells, citrate is reversibly isomerized to isocitrate as part of the Krebs cycle by the enzyme m-aconitase. However, the presence of extraordinarily high levels of zinc in prostate cells inhibits m-aconitase activity and allows for metabolically efficient citrate complexation in the PZ, which is later secreted as prostatic fluid. Because the Krebs cycle is not completed, typical ATP production is impaired, and the demand on glucose and aspartate by PZ cells is increased [29]. This process is summarized on the left side of Fig. 2.
In general, malignant transformation impairs normal mitochondrial functioning, requiring elevated glycolysis and lactic acid fermentation in the cytosol and production of lactate and alanine. This increases the glucose requirement and uptake in malignant cells and is known as the Warburg effect [33]. In contrast, and unique to PCa, is the inability of PZ epithelial cells to accumulate zinc. The resulting low zinc concentration allows m-aconitase to be active, citrate to be isomerized, and the previously truncated Krebs cycle to be completed, resulting in the production of an extra 24 ATP molecules through complete glucose oxidation and oxidative phosphorylation. Consequently, the increased level of oxidative phosphorylation produces more free radicals due to increased flux in the electron transport chain and accelerates neoplastic transformation [34]. In short, metabolism in malignant PCa cells is more efficient in producing bioenergy than is that in normal PZ epithelial cells, which is the opposite of most other malignant processes. This requires that further treatment strategies be investigated, because traditional methods may be ineffective, further complicating the prevention and treatment of PCa.
The metabolic transformation in PCa cells results in aninability to accumulate citrate, which is reflected in diminished levels in prostatic fluid [35]. Furthermore, this may be exacerbated by tumor growth impeding the luminal space [29]. This process is summarized on the right side of Fig. 2. Increased cell proliferation requires increased membraneogenesis and choline metabolites, reflected by increased choline and creatine levels in the PZ in PCa [36]. Although citrate, choline, and creatine in prostate biofluids and biopsies may be specific for prostate cancer, appropriate clinical utilization of their relationships to malignant progression is currently limited.
Analysis of metabolites within the prostate and biofluids has been undertaken by use of a variety of techniques. For samples in solution, the most commonly used methods are nuclear magnetic resonance (NMR) spectroscopy or gas and liquid chromatography-mass spectrometry (GC-MS) and liquid chromatography-mass spectrometry (LC-MS). Other methods of analysis include high resolution magic angle spinning (HR-MAS) NMR and magnetic resonance spectrometry (MRS) in tissue/in vivo and imaging techniques such as MRI or PET. Each of these methods helps to develop a snapshot of the metabolic state of the healthy or diseased prostate gland [37].
The basic principles of NMR spectroscopy applied in metabolomics are that a short radiofrequency (RF) pulse is used to excite energy levels of the nuclear spin of the protons within the sample. Different protons within different metabolites resonate at different resonance frequencies causing individual signals and signal patterns that are specific for each metabolite. The signal intensity is directly proportional to the metabolite concentration, resulting in the capacity for simultaneous quantification of multiple metabolites [38]. Using the same principle, HR-MAS NMR allows for simultaneous quantification of metabolites in solid-state tissue samples. This specific application has been extensively applied in vitro to tissues, either from fine needle aspiration or biopsy or surgical excision. This application is advantageous, because the analysis does not distort the tissue architecture, such that further histopathological analysis is not jeopardized.
Moreover, MRI when combined with NMR spectroscopy principles results in magnetic resonance spectroscopic imaging (MRSI), which allows for simultaneous quantification of metabolites in a section being imaged, allowing for in vivo application.
MS methods used with prostatic tissues and biofluids include LC-MS or GC-MS, which first separate metabolites in a sample by liquid or gas chromatography and then yield a mass spectrum of the resulting fractions [27]. MS methods have higher sensitivity than NMR methods but require more extensive sample preparation and can show chemical bias due to derivatization procedures. These methods have recently shown promise in PCa detection following urine analysis [22].
PET in combination with computerized tomography (CT) is widely used worldwide to produce three-dimensional images of visceral structures that are otherwise difficult to visualize. A positron-emitting radiolabeled tracer is introduced into the body and circulates while attached to an endogenous, biologically active molecule. Neoplastic cells preferentially metabolize the tracer, resulting in an accumulation and increased emission of ionizing radiation in malignant tissue. When applying PET to PCa metabolomics, different tracers are used to utilize varied alterations in cellular metabolic pathways to discriminate between healthy and malignant prostate tissue.
In the context of prostate physiology and quantification methods, metabolomic analysis has seen the identification of several promising metabolites for quantifying disease. The utility of selected metabolites and metabolite ratios identified to date for PCa diagnostics and prognostics is summarized in Table 2.
It is well known that, compared with healthy prostate, BPH tissue, and prostate-specific biofluids, PCa is characterized by low levels of citrate and polyamines (e.g., Spermine, myo-inositol), but high lactate, choline, and creatine levels [18,37,39-41]. PCa leads to altered zinc metabolism, resulting in diminished zinc, and consequently citrate, levels both in prostate tissue and in prostatic fluid [18,29,41-44]. Although citrate levels vary between PCa, BPH, and healthy PZ tissue, zinc levels remain relatively constant between samples from healthy, BPH, and prostatitis tissues, but are markedly reduced in PCa by up to more than 90% [45]. Moreover, levels of citrate and zinc in prostatic tissue and fluid are identical, indicating that prostatic fluid is representative of the metabolic status of the PZ. Such alterations to citrate and zinc levels imply that metabolic changes are essential to manifest malignant activities in neoplastic cells. Thus, it is understood that reduced citrate and zinc occur early in the malignant transformation to PCa [46]. This has been shown in vitro, as decreases in citrate exceed the volume of histopathologically identified cells, with the gap being the result of histologically unremarkable premalignant cells. However, these noncancerous cells contain genetic and metabolic alterations without changes in histological architecture, which is known as the 'field effect' in cancer biology [29]. On the other side of the cancer spectrum, both zinc and citrate are undetectable in poorly differentiated PCa tumors [47], indicating that the depletion of citrate and zinc is a progressive process.
Citrate is further reduced due to its use in increased membraneogenesis from malignant cell proliferation [29]. In addition, this process causes an increased requirement for choline and altered choline metabolism, the combination of which increases the concentration of choline-containing metabolites [41,48]. In this context, radiolabeled ethanolamine, a choline metabolite, has been implicated as a possible marker [49,50]. Lactate and alanine levels are attributed to the Warburg effect [25], as well as to tissue hypoxia during the sampling process, and as a ratio are proportional to tumor aggression and metastasis [51]. This is thought to be the result of PCa progression, but lactate has also been implicated in promoting metastasis via activation of molecular hypoxia-driven pathways, and upregulation of specific growth factors and proangiogenic genes promoting neovascularization, such as vascular endothelial growth factor (VEGF) [52,53]. The multifactorial nature of cancer has also been considered, with the influence of dietary factors such as polyunsaturated fatty acids (PUFA), having been investigated. It has been shown that omega-6 PUFA accumulate in malignant tissue samples but are undetectable in nonmalignant samples. Although this does render PUFA as an eligible biomarker, a lack of accumulation in the peri-tumor margins suggests that the accumulation is due to tumor growth and not to premalignant stages of cancer [54]. Choline, citrate, and spermine levels have been shown to correlate with Gleason score [18,44,55,56], whereas polyamine and sarcosine levels indicate PCa progression and tumor aggression [22,57]. Furthermore, sarcosine detected in the urine, via LC-MS/GC-MS, has been proposed as a method for detecting PCa and particularly the presence of metastases [22]. PCa progression to metastasis, as suggested by malignant metabolic changes shown on PET CT using 11C-choline, have been confirmed histologically in lymph nodes after radical prostatectomy and external beam radiotherapy [58]. The ratio of choline to creatine over citrate (CC/Ci) is also effective in evaluating clinical in vivo MRSI results [59,60].
Although single metabolites [52,55,61] and metabolite ratios [51] have been shown to distinguish between malignant and benign samples, they have been reported to lack sensitivity. Hence, the current consensus is that entire metabolic profiles are more sensitive in identifying and characterizing PCa [19,37,62]. This has been shown in PCa by using HR-MAS NMR to successfully identify areas of malignancy in prostate tissue [20,37,59,62]. When entire metabolomic profiles are evaluated by use of principal component analysis, differentiation of cancerous and histologically benign prostate tissue samples has been shown to be highly significant (p<0.0001) with an overall accuracy >98%. Moreover, some principal components had significant predictive powers in distinguishing between confined (T2) and invasive (T3) PCa [62]. Metabolomic profiling has also been shown to have an accuracy of 78% in predicting biochemical recurrence, which makes prognosis more individualized [63].
The contents of biofluids, when analyzed for various cytological and biochemical abnormalities, reflect homeostasis at a given moment. In the context of PCa detection, applicable biofluids include urine; blood; expressed prostatic secretion (EPS), which is collected in an immediately subsequent void of urine; and ejaculate. The possible preferred use of biofluids over biopsies, the current gold standard, is based on the less-invasive nature of sampling and that it can be undertaken frequently with only minimal risks of infection, bleeding, or discomfort. Although clinical use of 1H-NMR on EPS was initially limited by low-volume sample size, recent research has developed methods to make this method more effective, with the use of smaller probes to improve analysis [38].
Current markers in prostatic fluid, including EPS and ejaculate, relate to previous in vitro research. Diminished citrate and zinc levels in prostatic fluid have been shown to be representative of prostate tissue status in various disease states [18,29,42-45]. This is supported by the correlation of citrate and spermine depletion in prostatic fluid with Gleason score and citrate as an improvement on PSA in PCa detection [28,44].
However, false-positive results may occur with prostatitis-induced low citrate levels [64]. Zinc levels may be the differentiating factor, but further validation in prostatitis is required, given the various forms of this condition that may contribute to the inconclusive evidence [45,64]. In addition, false-positive results may be the consequence of pre-malignant transformation, which can only be determined subsequently when histology is the yardstick that is often normal in premalignant transformation, notwithstanding the relationships of high-grade prostatic intraepithelial neoplasia (HGPIN) and atypical findings to malignant transformation in a proportion of "at risk" patients [29]. Citrate production has also been shown to cease after radiotherapy and androgen deprivation therapy [65,66], indicating the need for a thorough history as well as further markers to assess response to treatment. False-negative results may occur with BPH-induced high levels of citrate and zinc, BPH being a common accompaniment of PCa. This scenario is less likely for the majority of PCa, however, because prostatic fluid findings mostly reflect the status of the PZ [29]. However, transitional zone cancers may be omitted from detection by using prostatic fluid, so further markers may be required for tumors arising in this part of the prostate.
The application of standard physiology, global metabolomic profiling, and identification of individual key metabolites is currently being investigated to develop an accurate, noninvasive, in vivo metabolomic analysis method to detect early PCa. Many different methods and approaches have been studied, some of which are summarized in Table 3.
In vivo, MRSI is currently the most widely used method of differentiating between healthy and malignant tissue by simultaneously imaging tissue structures and quantification of metabolites in situ [67,68]. Although rectal coils were used previously, most in vivo MRI is now "whole body." Similar to research in vitro, analysis was initially targeted at citrate and choline levels, which appeared to be promising [69]. As with prostatic fluid, analysis by MRSI of citrate and spermine depletion and choline elevation in prostatic tissue has been reported to correlate well with Gleason score [28,70,71]. However, this correlation is inconsistent and has been attributed to vagaries of sampling with biopsy needles (e.g., small foci of tumors, tissue heterogeneity, and Gleason score interpretation) [51], indicating a need for further and more comprehensive investigations. Citrate has also been stated to predict treatment responsiveness and relapse rates [27]. In addition, citrate quantification via MRSI has been reported to be superior to anatomic MRI in determining extracapsular extension and in reducing interobserver variability [72,73]. Furthermore, MRSI metabolomics can assist with prostate tissue sampling, being able to highlight areas of interest with a stated accuracy of over 90% [62].
Recent developments in PET for in vivo metabolomics use increased choline metabolism to visualize malignant cells with enhanced sensitivity. The radioactive PET marker 11C-choline is known to be metabolized in aggressive PCa and has been used to assist in visualizing bone metastases [74], as well as for the localization and staging of PCa [30]. This method of PET has been reported to exhibit an accuracy rate of 73% in patients with PSA levels higher than 3 ng/ml [75]. The 18F-labelled choline analogue has also been successful in detecting and staging PCa [30,76], whereas 11C-leucine and 14C-valine have been shown to be metabolized by PCa cells [77,78]. A more extensive review of choline-containing compounds as biomarkers is available from Glunde and Serkova [40].
However, in vivo MRI and PET are not without their limitations. MRI was initially limited in spectral resolution and was unable to measure individual metabolites. These limitations have been addressed recently, with the addition of diffusion-weighted imaging and dynamic contrast enhancement currently being trialled [19]. In addition, previous volume requirements for analysis have been addressed, with implementation of point-resolved spectroscopy (PRESS), which permits the analysis of smaller volumes (1 µl) [28].
PET limitations pertain predominantly to the markers used and the rates at which these accumulate in targeted tissues. Ongoing research is being undertaken to find better markers for accurate localization and staging of PCa.
Despite the already highlighted clinical need and scientific promise, all biomarkers that are identified require extensive validation before their potential can be realized in a clinical setting. An example is the recent debate about sarcosine, a putative biomarker for the early detection of PCa. In 2009, Sreekumar et al reported a study in which prostate tissue, serum, and post-digital rectal examination (DRE) urine were analyzed with LC-MS and GC-MS. They demonstrated sarcosine levels in tissue to be significantly correlated with prostate malignancy and metastatic potential. In addition, they showed that sarcosine can induce malignant changes when introduced to a prostate epithelial cell culture line [22]. Finally, they showed that sarcosine/alanine ratios in urinary sediment and sarcosine/creatinine ratios in urinary supernatants can discriminate modestly between PCa-positive and PCa-negative biopsy patients [22]. This last result of sarcosine as a potentially promising urinary biomarker created initial excitement [79,80]. However, in July 2010, García-Segura et al analyzed sarcosine levels in post-DRE urine samples in biopsy-confirmed patients and in patients with no evidence of malignancy by use of GC-MS [81]. They found that sarcosine normalized to creatinine had no association with tumor stage or grade and concluded that it was more beneficial than total PSA but was significantly worse than percentage free PSA. Since this second study was published, considerable debate has compared both studies and discussed their validity, with particular scrutiny paid to the study types (exploratory proof-of-concept versus comparative), composition of study cohorts, assay techniques, and sample types (urine sediment vs. urine supernatant) [82-89]. Independent confirmation seems now established that sarcosine levels in urine supernatants are not well correlated with cancer status [89,90]. Serum sarcosine levels also do not seem to correlate [82]. However, Sreekumar et al did confirm in an independent set of urine sediments that sarcosine/alanine ratios in urine sediments are correlated with biopsy status [85]. Thus, it appears that sarcosine levels in urine sediments and in tissue might be potential biomarker candidates, whereas levels in urinary supernatants or serum are not. It is currently unclear why a discrepancy exists between the results from urinary supernatant and those from sediment. In addition, in a new study, Jentzmik et al analyzed sarcosine levels in prostate tissue and showed that whereas sarcosine levels are 7% higher in malignant than in nonmalignant tissue, there is no correlation with tumor stage or grade [91].
In our opinion, the debate, although currently unresolved, has the potential to advance the field considerably. It highlights that in metabolomics close attention must be paid to the whole pipeline of study setup and design, sample analysis, metabolite normalization, data treatment, and statistical analysis, because a change in any of these parameters can contribute to different results even when based on the same raw data. In addition, it shows that although putative biomarkers may show promise in a laboratory, extensive investigation and trialling is required before these biomarker candidates will be suitable as an effective screening method for PCa. Interestingly, it has been highlighted that standardization in analysis and reporting of putative biomarkers needs to be established [83], as this will reduce premature and unnecessary hype among both PCa patients and practicing clinicians.
There is no doubt that the novel approach of metabolomic profiling of PCa for early detection and quantitative analysis of histopathology is exciting. However, in practice, a comprehensive correlation between metabolite data and clinicopathological findings needs to be established. One limitation to further development is the imprecision of PCa diagnosis in patients. A proposed solution to improving clinical and histologic diagnosis involves computer-aided analysis (CAIA) of prostate pathology slides, which has been shown to be more reliable than interobserver or intra-observer analysis [92].
Metabolomic analysis can detect precancerous changes, and the use of biofluids as noninvasive samples appears to be promising. In vivo methods in combination with CT and MRI that further substantiate evidence from biofluids will also provide visual guidance to improve our current diagnostic methods. In addition to indicating disease progression, metabolomics also has a potential role in the future in monitoring responses to therapeutic implementations [27], for example, in heralding or verifying chemo-resistance or radio-resistance [93], thus permitting a more rational integration of these forms of treatment with surgical interventions. Further to this, it is considered that the future for individualizing PCa diagnosis and treatment will involve a combination of metabonomics and genetic analyses to provide the most favorable treatment outcomes for individual patients.
Figures and Tables
FIG. 1
Summary of targets in the 'omic' era of analysis. The hierarchical levels of cellular organization involved in the progression from genotype to phenotype are shown. This expression of cellular phenotype is tightly regulated by feedback mechanisms, as shown above the progression. Through this process, various targets are available for analysis and opportunistic manipulation, as shown below the progression.
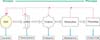
FIG. 2
Summary of peripheral prostate physiology in health (left) and disease (right). Healthy peripheral prostate physiology involves citrate accumulation via the zinc-dependent inhibition of m-aconitase, resulting in reduced ATP production via the Krebs cycle and a greater dependence on glucose and aspartate for ATP. In malignancy, peripheral prostate cells lose their ability to accumulate zinc, resulting in isomerization of citrate via m-aconitase for metabolism in the Krebs cycle and reduced citrate accumulation and production.
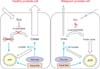
FIG. 3
Relationships between different quantification methods. The relationships between different quantification methods are shown. The basic techniques of quantification are listed on the left side, their relationships and combinations are shown in the middle of the diagram, and the result of these relationships is shown on the right side. These are the principal methods of quantification in prostate cancer (PCa) metabolomics. LC-MS: liquid chromatography mass spectrometry, GC-MS: gas chromatography mass spectrometry, NMR: nuclear magnetic resonance, MRI: magnetic resonance imaging, MRSI: magnetic resonance spectroscopic imaging, PET: positron emission tomography.
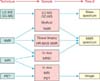
TABLE 1
Analytical quantification methods for metabolites
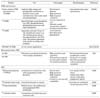
Different analytical methods used in metabolomics have their own advantages and disadvantages that predispose them for different applications as summarized in this table. NMR: nuclear magnetic resonance, LC-MS: liquid chromatography mass spectrometry, GC-MS: gas chromatography mass spectrometry, FDG: fluorodeoxyglucose, PCa: prostate cancer
References
1. Park SK, Sakoda LC, Kang D, Chokkalingam AP, Lee E, Shin HR, et al. Rising prostate cancer rates in South Korea. Prostate. 2006. 66:1285–1291.
2. Jemal A, Siegel R, Xu J, Ward E. Cancer statistics, 2010. CA Cancer J Clin. 2010. 60:277–300.
3. Marugame T, Mizuno S. Comparison of prostate cancer mortality in five countries: France, Italy, Japan, UK and USA from the WHO mortality database (1960-2000). Jpn J Clin Oncol. 2005. 35:690–691.
4. Sim HG, Cheng CW. Changing demography of prostate cancer in Asia. Eur J Cancer. 2005. 41:834–845.
5. Yang S Y, Adelstein J, Kassis AI. Putative molecular signatures for the imaging of prostate cancer. Expert Rev Mol Diagn. 2010. 10:65–74.
6. Narain V, Cher ML, Wood DP Jr. Prostate cancer diagnosis, staging and survival. Cancer Metastasis Rev. 2002. 21:17–27.
7. Lin K, Lipsitz R, Miller T, Janakiraman S. Benefits and harms of prostate-specific antigen screening for prostate cancer: an evidence update for the U.S. Preventive Services Task Force. Ann Intern Med. 2008. 149:192–199.
8. Crawford ED. PSA testing: what is the use? Lancet. 2005. 365:1447–1449.
9. Thompson IM, Pauler DK, Goodman PJ, Tangen CM, Lucia MS, Parnes HL, et al. Prevalence of prostate cancer among men with a prostate-specific antigen level < or =4.0 ng per milliliter. N Engl J Med. 2004. 350:2239–2246.
10. Pienta KJ. Critical appraisal of prostate-specific antigen in prostate cancer screening: 20 years later. Urology. 2009. 73:5 Suppl. S11–S20.
11. Schröder FH, Hugosson J, Roobol MJ, Tammela TL, Ciatto S, Nelen V, et al. Screening and prostate-cancer mortality in a randomized European study. N Engl J Med. 2009. 360:1320–1328.
12. Barry MJ. Screening for prostate cancer--the controversy that refuses to die. N Engl J Med. 2009. 360:1351–1354.
13. Bensalah K, Lotan Y, Karam JA, Shariat SF. New circulating biomarkers for prostate cancer. Prostate Cancer Prostatic Dis. 2008. 11:112–120.
14. Veltri RW, Miller MC, O'dowd GJ, Partin AW. Impact of age on total and complexed prostate-specific antigen cutoffs in a contemporary referral series of men with prostate cancer. Urology. 2002. 60:4 Suppl 1. 47–52.
15. Fitzpatrick JM. The natural history of benign prostatic hyperplasia. BJU Int. 2006. 97:Suppl 2. 3–6.
16. Stenman UH, Leinonen J, Zhang WM, Finne P. Prostate-specific antigen. Semin Cancer Biol. 1999. 9:83–93.
17. Hansel DE, DeMarzo AM, Platz EA, Jadallah S, Hicks J, Epstein JI, et al. Early prostate cancer antigen expression in predicting presence of prostate cancer in men with histologically negative biopsies. J Urol. 2007. 177:1736–1740.
18. Serkova NJ, Gamito EJ, Jones RH, O'Donnell C, Brown JL, Green S, et al. The metabolites citrate, myo-inositol, and spermine are potential age-independent markers of prostate cancer in human expressed prostatic secretions. Prostate. 2008. 68:620–628.
19. DeFeo EM, Cheng LL. Characterizing human cancer metabolomics with ex vivo 1H HRMAS MRS. Technol Cancer Res Treat. 2010. 9:381–391.
20. Hricak H, Choyke PL, Eberhardt SC, Leibel SA, Scardino PT. Imaging prostate cancer: a multidisciplinary perspective. Radiology. 2007. 243:28–53.
21. Clarke RA, Schirra HJ, Catto JW, Lavin MF, Gardiner RA. Markers for detection of prostate cancer. Cancers. 2010. 2:1125–1154.
22. Sreekumar A, Poisson LM, Rajendiran TM, Khan AP, Cao Q, Yu J, et al. Metabolomic profiles delineate potential role for sarcosine in prostate cancer progression. Nature. 2009. 457:910–914.
23. Nicholson JK, Lindon JC, Holmes E. 'Metabonomics': understanding the metabolic responses of living systems to pathophysiological stimuli via multivariate statistical analysis of biological NMR spectroscopic data. Xenobiotica. 1999. 29:1181–1189.
24. Costello LC, Franklin RB. The clinical relevance of the metabolism of prostate cancer; zinc and tumor suppression: connecting the dots. Mol Cancer. 2006. 5:17.
25. Griffin JL, Shockcor JP. Metabolic profiles of cancer cells. Nat Rev Cancer. 2004. 4:551–561.
26. Abate-Shen C, Shen MM. Diagnostics: the prostate-cancer metabolome. Nature. 2009. 457:799–800.
27. Spratlin JL, Serkova NJ, Eckhardt SG. Clinical applications of metabolomics in oncology: a review. Clin Cancer Res. 2009. 15:431–440.
28. Serkova NJ, Spratlin JL, Eckhardt SG. NMR-based metabolomics: translational application and treatment of cancer. Curr Opin Mol Ther. 2007. 9:572–585.
29. Costello LC, Franklin RB. Prostatic fluid electrolyte composition for the screening of prostate cancer: a potential solution to a major problem. Prostate Cancer Prostatic Dis. 2009. 12:17–24.
30. Teahan O, Bevan CL, Waxman J, Keun HC. Metabolic signatures of malignant progression in prostate epithelial cells. Int J Biochem Cell Biol. 2010. Epub ahead of print.
31. Lynch MJ, Masters J, Pryor JP, Lindon JC, Spraul M, Foxall PJ, et al. Ultra high field NMR spectroscopic studies on human seminal fluid, seminal vesicle and prostatic secretions. J Pharm Biomed Anal. 1994. 12:5–19.
32. Liney GP, Turnbull LW, Lowry M, Turnbull LS, Knowles AJ, Horsman A. In vivo quantification of citrate concentration and water T2 relaxation time of the pathologic prostate gland using 1H MRS and MRI. Magn Reson Imaging. 1997. 15:1177–1186.
33. Warburg O. On the origin of cancer cells. Science. 1956. 123:309–314.
34. Dakubo GD, Parr RL, Costello LC, Franklin RB, Thayer RE. Altered metabolism and mitochondrial genome in prostate cancer. J Clin Pathol. 2006. 59:10–16.
35. Sciarra A, Panebianco V, Ciccariello M, Salciccia S, Lisi D, Osimani M, et al. Magnetic resonance spectroscopic imaging (1H-MRSI) and dynamic contrast-enhanced magnetic resonance (DCE-MRI): pattern changes from inflammation to prostate cancer. Cancer Invest. 2010. 28:424–432.
36. Noworolski SM, Vigneron DB, Chen AP, Kurhanewicz J. Dynamic contrast-enhanced MRI and MR diffusion imaging to distinguish between glandular and stromal prostatic tissues. Magn Reson Imaging. 2008. 26:1071–1080.
37. Cheng LL, Burns MA, Taylor JL, He W, Halpern EF, McDougal WS, et al. Metabolic characterization of human prostate cancer with tissue magnetic resonance spectroscopy. Cancer Res. 2005. 65:3030–3034.
38. Serkova NJ, Freund AS, Brown JL, Kominsky DJ. Use of the 1-mm micro-probe for metabolic analysis on small volume biological samples. J Cell Mol Med. 2009. 13:1933–1941.
39. Costello LC, Franklin RB, Narayan P. Citrate in the diagnosis of prostate cancer. Prostate. 1999. 38:237–245.
40. Glunde K, Serkova NJ. Therapeutic targets and biomarkers identified in cancer choline phospholipid metabolism. Pharmacogenomics. 2006. 7:1109–1123.
41. Lynch MJ, Nicholson JK. Proton MRS of human prostatic fluid: correlations between citrate, spermine, and myo-inositol levels and changes with disease. Prostate. 1997. 30:248–255.
42. Cooper JF, Imfeld H. The role of citric acid in the physiology of the prostate: a preliminary report. J Urol. 1959. 81:157–164.
43. Averna TA, Kline EE, Smith AY, Sillerud LO. A decrease in 1H nuclear magnetic resonance spectroscopically determined citrate in human seminal fluid accompanies the development of prostate adenocarcinoma. J Urol. 2005. 173:433–438.
44. Kline EE, Treat EG, Averna TA, Davis MS, Smith AY, Sillerud LO. Citrate concentrations in human seminal fluid and expressed prostatic fluid determined via 1H nuclear magnetic resonance spectroscopy outperform prostate specific antigen in prostate cancer detection. J Urol. 2006. 176:2274–2279.
45. Zaichick VY, Sviridova TV, Zaichick SV. Zinc concentration in human prostatic fluid: normal, chronic prostatitis, adenoma and cancer. Int Urol Nephrol. 1996. 28:687–694.
46. Cooper JF, Farid I. The role of citric acid in the physiology of the prostate. 3. lactate/citrate ratios in benign and malignant prostatic homogenates as an index of prostatic malignancy. J Urol. 1964. 92:533–536.
47. Kurhanewicz J, Dahiya R, Macdonald JM, Chang LH, James TL, Narayan P. Citrate alterations in primary and metastatic human prostatic adenocarcinomas: 1H magnetic resonance spectroscopy and biochemical study. Magn Reson Med. 1993. 29:149–157.
48. Cornel EB, Smits GA, Oosterhof GO, Karthaus HF, Deburyne FM, Schalken JA, et al. Characterization of human prostate cancer, benign prostatic hyperplasia and normal prostate by in vitro 1H and 31P magnetic resonance spectroscopy. J Urol. 1993. 150:2019–2024.
49. Mintz A, Wang L, Ponde DE. Comparison of radiolabeled choline and ethanolamine as probe for cancer detection. Cancer Biol Ther. 2008. 7:742–747.
50. Podo F. Tumour phospholipid metabolism. NMR Biomed. 1999. 12:413–439.
51. van Asten JJ, Cuijpers V, Hulsbergen-van de Kaa C, Soede-Huijbregts C, Witjes JA, Verhofstad A, et al. High resolution magic angle spinning NMR spectroscopy for metabolic assessment of cancer presence and Gleason score in human prostate needle biopsies. MAGMA. 2008. 21:435–442.
52. Tessem MB, Swanson MG, Keshari KR, Albers MJ, Joun D, Tabatabai ZL, et al. Evaluation of lactate and alanine as metabolic biomarkers of prostate cancer using 1H HR-MAS spectroscopy of biopsy tissues. Magn Reson Med. 2008. 60:510–516.
53. Mori R, Dorff TB, Xiong S, Tarabolous CJ, Ye W, Groshen S, et al. The relationship between proangiogenic gene expression levels in prostate cancer and their prognostic value for clinical outcomes. Prostate. 2010. 70:1692–1700.
54. Stenman K, Hauksson JB, Gröbner G, Stattin P, Bergh A, Riklund K. Detection of polyunsaturated omega-6 fatty acid in human malignant prostate tissue by 1D and 2D high-resolution magic angle spinning NMR spectroscopy. MAGMA. 2009. 22:327–331.
55. Swanson MG, Zektzer AS, Tabatabai ZL, Simko J, Jarso S, Keshari KR, et al. Quantitative analysis of prostate metabolites using 1H HR-MAS spectroscopy. Magn Reson Med. 2006. 55:1257–1264.
56. Kurhanewicz J, Vigneron DB, Nelson SJ. Three-dimensional magnetic resonance spectroscopic imaging of brain and prostate cancer. Neoplasia. 2000. 2:166–189.
57. Swanson MG, Vigneron DB, Tabatabai ZL, Males RG, Schmitt L, Carroll PR, et al. Proton HR-MAS spectroscopy and quantitative pathologic analysis of MRI/3D-MRSI-targeted postsurgical prostate tissues. Magn Reson Med. 2003. 50:944–954.
58. Schilling D, Schlemmer HP, Wagner PH, Bottcher P, Merseburger AS, Aschoff P, et al. Histological verification of 11C-choline-positron emission/computed tomography-positive lymph nodes in patients with biochemical failure after treatment for localized prostate cancer. BJU Int. 2008. 102:446–451.
59. Mazaheri Y, Shukla-Dave A, Hricak H, Fine SW, Zhang J, Inurrigarro G, et al. Prostate cancer: identification with combined diffusion-weighted MR imaging and 3D 1H MR spectroscopic imaging--correlation with pathologic findings. Radiology. 2008. 246:480–488.
60. Scheenen TW, Heijmink SW, Roell SA, Hulsbergen-Van de Kaa CA, Knipscheer BC, Witjes JA, et al. Three-dimensional proton MR spectroscopy of human prostate at 3 T without endorectal coil: feasibility. Radiology. 2007. 245:507–516.
61. Swanson MG, Keshari KR, Tabatabai ZL, Simko JP, Shinohara K, Carroll PR, et al. Quantification of choline- and ethanolamine-containing metabolites in human prostate tissues using 1H HR-MAS total correlation spectroscopy. Magn Reson Med. 2008. 60:33–40.
62. Wu CL, Jordan KW, Ratai EM, Sheng J, Adkins CB, Defeo EM, et al. Metabolomic imaging for human prostate cancer detection. Sci Transl Med. 2010. 2:16ra8.
63. Maxeiner A, Adkins CB, Zhang Y, Taupitz M, Halpern EF, McDougal WS, et al. Retrospective analysis of prostate cancer recurrence potential with tissue metabolomic profiles. Prostate. 2010. 70:710–717.
64. Kavanagh JP, Darby C, Costello CB. The response of seven prostatic fluid components to prostatic disease. Int J Androl. 1982. 5:487–496.
65. Menard C, Smith IC, Somorjai RL, Leboldus L, Patel R, Littman C, et al. Magnetic resonance spectroscopy of the malignant prostate gland after radiotherapy: a histopathologic study of diagnostic validity. Int J Radiat Oncol Biol Phys. 2001. 50:317–323.
66. Mueller-Lisse UG, Swanson MG, Vigneron DB, Hricak H, Bessette A, Males RG, et al. Time-dependent effects of hormone-deprivation therapy on prostate metabolism as detected by combined magnetic resonance imaging and 3D magnetic resonance spectroscopic imaging. Magn Reson Med. 2001. 46:49–57.
67. Fass L. Imaging and cancer: a review. Mol Oncol. 2008. 2:115–152.
68. Kurhanewicz J, Vigneron DB. Advances in MR spectroscopy of the prostate. Magn Reson Imaging Clin N Am. 2008. 16:697–710.
69. Hom JJ, Coakley FV, Simko JP, Lu Y, Qayyum A, Westphalen AC, et al. High-grade prostatic intraepithelial neoplasia in patients with prostate cancer: MR and MR spectroscopic imaging features--initial experience. Radiology. 2007. 242:483–489.
70. Takyi EE, Fuller DJ, Donaldson LJ, Thomas GH. Deoxyribonucleic acid and polyamine synthesis in rat ventral prostrate. Effects of age of the intact rat and androgen stimulation of the castrated rat with testosterone, 5 alpha-dihydrotestosterone and 5 alpha-androstane-3 beta, 17 beta-diol. Biochem J. 1977. 162:87–97.
71. Kurhanewicz J, Swanson MG, Nelson SJ, Vigneron DB. Combined magnetic resonance imaging and spectroscopic imaging approach to molecular imaging of prostate cancer. J Magn Reson Imaging. 2002. 16:451–463.
72. Scheidler J, Hricak H, Vigneron DB, Yu KK, Sokolov DL, Huang LR, et al. Prostate cancer: localization with three-dimensional proton MR spectroscopic imaging--clinicopathologic study. Radiology. 1999. 213:473–480.
73. Yu KK, Scheidler J, Hricak H, Vigneron DB, Zaloudek CJ, Males RG, et al. Prostate cancer: prediction of extracapsular extension with endorectal MR imaging and three-dimensional proton MR spectroscopic imaging. Radiology. 1999. 213:481–488.
74. Hara T, Kosaka N, Kishi H. PET imaging of prostate cancer using carbon-11-choline. J Nucl Med. 1998. 39:990–995.
75. Krause BJ, Souvatzoglou M, Tuncel M, Herrmann K, Buck AK, Praus C, et al. The detection rate of [11C]choline-PET/CT depends on the serum PSA-value in patients with biochemical recurrence of prostate cancer. Eur J Nucl Med Mol Imaging. 2008. 35:18–23.
76. Price DT, Coleman RE, Liao RP, Robertson CN, Polascik TJ, DeGrado TR. Comparison of [18 F]fluorocholine and [18 F]fluorodeoxyglucose for positron emission tomography of androgen dependent and androgen independent prostate cancer. J Urol. 2002. 168:273–280.
77. Kirikae M, Diksic M, Yamamoto YL. Quantitative measurements of regional glucose utilization and rate of valine incorporation into proteins by double-tracer autoradiography in the rat brain tumor model. J Cereb Blood Flow Metab. 1989. 9:87–95.
78. Ishiwata K, Kubota K, Murakami M, Kubota R, Senda M. A comparative study on protein incorporation of L-[methyl-3H]methionine, L-[1-14C]leucine and L-2-[18F]fluorotyrosine in tumor bearing mice. Nucl Med Biol. 1993. 20:895–899.
79. Anderson RU, Fair WR. Physical and chemical determinations of prostatic secretion in benign hyperplasia, prostatitis, and adenocarcinoma. Invest Urol. 1976. 14:137–140.
80. van der Graaf M, Schipper RG, Oosterhof GO, Schalken JA, Verhofstad AA, Heerschap A. Proton MR spectroscopy of prostatic tissue focused on the detection of spermine, a possible biomarker of malignant behavior in prostate cancer. MAGMA. 2000. 10:153–159.
81. García-Segura JM, Sánchez-Chapado M, Ibarburen C, Viaño J, Angulo JC, González J, et al. In vivo proton magnetic resonance spectroscopy of diseased prostate: spectroscopic features of malignant versus benign pathology. Magn Reson Imaging. 1999. 17:755–765.
82. Struys EA, Heijboer AC, van Moorselaar J, Jakobs C, Blankenstein MA. Serum sarcosine is not a marker for prostate cancer. Ann Clin Biochem. 2010. 47:282.
83. Schalken JA. Is urinary sarcosine useful to identify patients with significant prostate cancer? The trials and tribulations of biomarker development. Eur Urol. 2010. 58:19–20.
84. Stephan C, Jentzmik F, Jung K. Reply from Authors re: Jack A. Schalken. Is urinary sarcosine useful to identify patients with significant prostate cancer? The trials and tribulations of biomarker development. Eur Urol 2010;58:19-20. Eur Urol. 2010. 58:20–21.
85. Sreekumar A, Poisson LM, Rajendiran TM, Khan AP, Cao Q, Yu J, et al. Re: Florian Jentzmik, Carsten Stephan, Kurt Miller, et al. Sarcosine in urine after digital rectal examination fails as a marker in prostate cancer detection and identification of aggressive tumours. Eur Urol 2010;58:12-8. Eur Urol. 2010. 58:e29–e30.
86. Jentzmik F, Stephan C, Jung K. Reply to Arun Sreekumar, Laila M. Poisson, Thekkelnaycke M. Rajendiran, et al.'s Letter to the Editor re: Florian Jentzmik, Carsten Stephan, Kurt Miller, et al. Sarcosine in Urine after Digital Rectal Examination Fails as a Marker in Prostate Cancer Detection and Identification of Aggressive Tumours. Eur Urol 2010;58:12-8. Eur Urol. 2010. 58:e31–e32.
87. Hewavitharana AK. Re: Florian Jentzmik, Carsten Stephan, Kurt Miller, et al. Sarcosine in urine after digital rectal examination fails as a marker in prostate cancer detection and identification of aggressive tumours. Eur Urol 2010;58:12-8. Eur Urol. 2010. 58:e39–e40.
88. Jentzmik F, Stephan C, Jung K. Reply to Amitha K Hewavitharana's Letter to the Editor re: Florian Jentzmik, Carsten Stephan, Kurt Miller, et al. Sarcosine in Urine After Digital Examination Fails as a Marker in Prostate Cancer Detection and Identification of Aggressive Tumours. Eur Urol 2010;58:12-8. Eur Urol. 2010. 58:e41–e42.
89. Colleselli D, Stenzl A, Schwentner C. Re: Florian Jentzmik, Carsten Stephan, Kurt Miller, et al. Sarcosine in urine after digital rectal examination fails as a marker in prostate cancer detection and identification of aggressive tumours. Eur Urol 2010; 58:12-8. Eur Urol. 2010. 58:e51.
90. Jentzmik F, Stephan C, Miller K, Schrader M, Erbersdobler A, Kristiansen G, et al. Sarcosine in urine after digital rectal examination fails as a marker in prostate cancer detection and identification of aggressive tumours. Eur Urol. 2010. 58:12–18.
91. Jentzmik F, Stephan C, Lein M, Miller K, Kamlage B, Bethan B, et al. Sarcosine in prostate cancer tissue is not a differential metabolite for prostate cancer aggressiveness and biochemical progression. J Urol. 2010. Epub ahead of print.
92. Burns MA, He W, Wu CL, Cheng LL. Quantitative pathology in tissue MR spectroscopy based human prostate metabolomics. Technol Cancer Res Treat. 2004. 3:591–598.
93. Mahdy AE, Cheng JC, Li J, Elojeimy S, Meacham WD, Turner LS, et al. Acid ceramidase upregulation in prostate cancer cells confers resistance to radiation: AC inhibition, a potential radiosensitizer. Mol Ther. 2009. 17:430–438.
94. Umbehr M, Bachmann LM, Held U, Kessler TM, Sulser T, Weishaupt D, et al. Combined magnetic resonance imaging and magnetic resonance spectroscopy imaging in the diagnosis of prostate cancer: a systematic review and meta-analysis. Eur Urol. 2009. 55:575–590.
95. Cheng LL, Wu C, Smith MR, Gonzalez RG. Non-destructive quantitation of spermine in human prostate tissue samples using HRMAS 1H NMR spectroscopy at 9.4 T. FEBS Lett. 2001. 494:112–116.
96. Jadvar H. Molecular imaging of prostate cancer: a concise synopsis. Mol Imaging. 2009. 8:56–64.
97. Kurhanewicz J, Vigneron DB, Nelson SJ, Hricak H, MacDonald JM, Konety B, et al. Citrate as an in vivo marker to discriminate prostate cancer from benign prostatic hyperplasia and normal prostate peripheral zone: detection via localized proton spectroscopy. Urology. 1995. 45:459–466.
98. Heerschap A, Jager GJ, van der Graaf M, Barentsz JO, de la Rosette JJ, Oosterhof GO, et al. In vivo proton MR spectroscopy reveals altered metabolite content in malignant prostate tissue. Anticancer Res. 1997. 17:1455–1460.
99. Kumar R, Nayyar R, Kumar V, Gupta NP, Hemal AK, Jagannathan NR, et al. Potential of magnetic resonance spectroscopic imaging in predicting absence of prostate cancer in men with serum prostate-specific antigen between 4 and 10 ng/ml: a follow-up study. Urology. 2008. 72:859–863.
100. Kurhanewicz J, Vigneron DB, Hricak H, Narayan P, Carroll P, Nelson SJ. Three-dimensional H-1 MR spectroscopic imaging of the in situ human prostate with high (0.24-0.7-cm3) spatial resolution. Radiology. 1996. 198:795–805.