Abstract
Purpose
We sought to maximize the antitumor effect of an anticancer vaccine based on genetically modified endothelial cells by combining it with the platelet-derived growth factor receptor inhibitor imatinib.
Materials and Methods
Human umbilical vein endothelial cells (HUVECs) were infected with 10 MOI of Ad-CMV-mGMCSF to make anticancer vaccines. One million mouse bladder cancer cells (MBT-2) were subcutaneously inoculated in C3H mice. The experimental groups included the following: Group 1 (phosphate-buffered saline), Group 2 (anticancer vaccine and GM-CSF), Group 3 (imatinib), and Group 4 (anticancer vaccine, GM-CSF, and imatinib). Tumor growth and body weight were measured weekly. At 4 weeks, the tumors were immunostained with anti-CD31, and microvessel density (MVD) was measured. To evaluate the immunological mechanism of each treatment, flow cytometry analysis of activated CD4 and CD8 cells was performed.
Results
At 4 weeks, the mean body weight of each group, excluding the extracted tumor weight, was not significantly different. Since week 3, the mean tumor volume in Group 4 was the smallest among the treatment groups (p<0.05), and a synergistic suppressive effect on tumor volume was observed in Group 4. The MVD in Group 4 was the most suppressed among the treatment groups (p<0.05), and a synergistic anti-angiogenic effect was observed. Flow cytometry analysis revealed that activated CD4+ and CD8+ cells increased in Group 2 and decreased in Group 3 compared with the other groups.
The chemotherapeutic methotrexate, vinblastine, Adriamycin, and cisplatin (M-VAC) and gemcitabine, cisplatin (GP) regimens have been considered standard chemotoxic management for advanced bladder cancer because of the relatively high response rate observed during the early period. However, the long-term prognosis with the above regimens is poor, with a median survival period of 12 to 13 months and a long-term survival rate of less than 5% [1,2]. Moreover, the side effects of standard cytotoxic chemotherapy cannot be ignored. Therefore, a new treatment modality is needed to achieve better anti-tumor effects with fewer side effects.
Preclinical and clinical studies of cancer vaccines, such as viral vaccines, DNA vaccines using anti-tumor antigens and tumor-specific genes, genetically modified tumor vaccines, and vaccines that use dendritic cells, have been performed. With the practice of instilling Bacillus Calmette-Guérin (BCG) intravesically to induce a local immune reaction in superficial bladder cancer [3], the induction of a T-cell-mediated immune reaction specific to the cancer cell and activation of innate immunity, including natural killer cells, can be considered reasonable alternatives in the treatment of advanced bladder cancer. Cancer cells escape from immune surveillance via several mechanisms, including cancer-specific antigen alterations, suppression of molecules that present the antigen for MHC I, and active secretion of immunosuppressive substances [4]. Therefore, bypassing the cancer cells themselves and targeting the stromal cells that support the cancer cells may be a novel approach to overcoming the escape mechanism of cancer cells. Wei et al reported that a vaccine that targets the tumor neovasculature has anti-tumor and preventive effects by activating the antibody reaction to angiogenetic-specific proteins, such as vascular endothelial growth factor receptor (VEGFR)-2 and αvβ3 integrin [5]. Since then, targeting stromal cells and the neovasculature as an approach to anti-tumor treatment has become an ongoing area of research [6-8].
Furthermore, various signal transduction pathways, including the epidermal growth factor receptor (EGFR) pathway, vascular endothelial growth factor (VEGF) pathway, and Ras/mitogen-activated protein kinase (Ras/MAPK) pathway, play significant roles in carcinogenesis and the progression of bladder cancer. Efforts to apply various tyrosine kinase inhibitors or monoclonal antibodies to the treatment of bladder cancer have been ongoing [9-11]. The VEGFR-2 signal transduction pathway is known as a central component of tumor neoangiogenesis, and the role of the platelet-derived growth factor (PDGF) pathway in the angiogenesis of various tumors has also been shown in several studies [12,13]. In the present study, we evaluated anti-tumor effects by inhibiting neoangiogenesis by use of a combination of a genetically modified endothelial cell vaccine and imatinib, which inhibits PDGF receptor (PDGFR) inhibitor.
Human umbilical vein endothelial cells (HUVECs), of less than 20 passages, were purchased from the American Type Culture Collection (ATCC) for the production of an anticancer vaccine and were grown in F-12K medium (ATCC, Manassas, VA, USA) supplemented with 10% fetal bovine serum (Gibco, Grand Island, NY, USA), 0.1 mg/ml heparin (Sigma-Aldrich, St. Louis, MO, USA), and 0.03 mg/ml endothelial cell growth supplement (Sigma-Aldrich) in a humidified atmosphere at 37℃ with 5% CO2.
For the bladder cancer cell line, murine bladder tumor-2 (MBT-2) cells induced by oral administration of N-[4-(5-nitro-2-furyl)-2-thiazolyl]-foramide in C3H/HeN mice, provided by Prof. W.J. Kim, Department of Urology, Chungbuk National University of Medicine, were used. The MBT-2 cells were grown in RPMI-1640 medium supplemented with 10% heat-inactivated fetal bovine serum (Gibco), 50 units/ml penicillin, and 50 µg/ml streptomycin in a humidified atmosphere at 37℃ with 5% CO2.
Adenovirus transfected with murine GM-CSF (Ad-CMVmGMCSF), provided by Dr. L.W. Chung, Department of Urology, University of Virginia, was used to produce the anticancer vaccine as previously reported [8]. 911 cells were infected with 10 multiplicity of infection (MOI) of virus and were kept frozen. Some of the 911 cells were cultured by using Cell Factory (Thermoscientific, Wilmington, DE, USA). When cell confluence reached 80%, the frozen 911 cells were thawed three times, followed by centrifugation, and the supernatant was injected into the cultured 911 cells. When a cytopathic effect was observed in more than 90% of the cells, the cells were collected and kept frozen. High-concentration virus was refined by using CsCl gradient centrifugation.
HUVECs were plated in a six-well plate at a density of 2×106 cells/well. Twenty-four hours after plating, the incubation medium was replaced, and HUVECs were infected with 10 MOI of Ad-CMV-mGMCSF. Twelve hours after infection, infected HUVECs (anticancer vaccines) were collected and washed with phosphate-buffered saline (PBS) three times.
After approval from the Seoul National University Hospital Institutional Animal Care and Use Committee (SNUH IACUC), the care of 4-week-old male C3H/HeN mice was performed according to the Guidelines for the Care and Use of Laboratory Animals in a Biological Safety Level 3 Laboratory. The mice were inoculated with 1×106 MBT-2 cells diluted with 100 µl PBS subcutaneously. After 3 weeks, the mice were randomly assigned into four groups (n=3 per group): Group 1 (PBS injection group), Group 2 (anticancer vaccine+GM-CSF injection group), Group 3 (imatinib injection group), and Group 4 (anticancer vaccine+imatinib +GM-CSF injection group). In Group 1, 100 µl PBS was injected intraperitoneally weekly. In Group 2, anticancer vaccines and 1 µg of recombinant mouse GM-CSF diluted with 100 µl PBS were injected intraperitoneally weekly. In Group 3, 50 mg/kg imatinib (LC Laboratories, Woburn, MA, USA) diluted with 100 µl PBS was injected intraperitoneally daily. In Group 4, anticancer vaccines, recombinant mouse GM-CSF, and imatinib were injected in the same manner as above. To evaluate the safety of each treatment modality, we checked the body weight of the mice weekly. To evaluate the anti-tumor effect of each treatment modality, the tumor volume was measured weekly. To calculate the tumor volume, one observer measured the long and short diameter of the tumor and calculated the tumor volume by use of the following formula:
long diameter of tumor × (short diameter of tumor)2×0.5.
After 4 weeks of treatment, the animals were euthanized with 5 mg/kg Zoletil, and the tumors and spleens were harvested.
The extracted spleen was crushed by using a tissue grinder and was washed with RPMI-1640 medium. After filtering the splenocytes in a 75 µm cell strainer, the filtered cells were centrifuged at 1,200 rpm for 10 min, and the supernatant was discarded. The remaining cell pellets were washed with PBS and resuspended and cultured in 1 ml RBC lysis buffer (Sigma-Aldrich, St. Louis, MO, USA). Cultured cells were counted to 1×106 with an automated counter (Coulter Corporation, Miami, FL, USA) and suspended in 50 µl FACS buffer (1× HBSS, 1.5% FBS, 1x sodium azide). The suspended cells were immunohistochemically stained with anti-mouse CD 69-PE, CD8a-FITC, and CD4-FITC (BD Biosciences, Franklin Lakes, NJ, USA) at 0℃ for 20 to 40 min, followed by washing twice with PBS. After cell fixation using 400 µl of 3% formaldehyde, flow cytometry was performed with a FACSCalibur™ device (BD Biosciences).
The tumors were fixed in 10% neutral buffered formalin and embedded in paraffin. Tissue sections were cut to 4 µm thickness and immunohistochemically stained with CD31 (Santa Cruz Biotechnology, Santa Cruz, CA, USA). According to Gasparini's criteria, the mean microvessel count of the 20 randomly selected areas was defined as the microvessel density (MVD), which was expressed as the absolute number of microvessels per 0.74 mm2 (200× field) [14]. To reduce observer-related variation, the counting of the microvessels was performed with a computer image analyzer.
SPSS ver. 17.0 (SPSS Inc., Chicago, IL, USA) was used for the statistical analysis. The results are expressed as means and standard deviations. Differences between treatment groups were examined by using the Kruskal-Wallis test and Mann-Whitney U test. Values of p<0.05 were considered statistically significant. To evaluate the combination effect of the vaccine and imatinib, the Chou and Talalay combination index was calculated by using CalcuSyn software (Biosoft, Cambridge, United Kingdom) [15]. If the combination index was less than 1, then we judged it as having a synergistic effect. If it was equal to 1, then we judged it as having an additive effect. If it was greater than 1, then we judged it as having an antagonistic effect.
Before the treatment, the mean body weight of each treatment group showed no significant difference (Groups 1-4, respectively: 26.6±0.6 g, 26.6±0.4 g, 26.9±0.6 g, and 26.7±0.8 g; p=0.795). After 2 weeks of treatment, the mean body weights of Groups 2, 3, and 4 were less than that of Group 1 (Fig. 1). However, when the body weight without the mass of the extracted tumor was compared at week 4, no significant differences were observed between the groups (Groups 1-4, respectively: 24.8±0.7 g, 19.1±1.9 g, 20.7±2.1 g, and 21.6±0.9 g; p=0.412) (Fig. 2). Therefore, we assumed that no significant weight loss was caused by the anticancer vaccine or imatinib.
At 3 weeks after tumor inoculation, the mean tumor volumes in Groups 1 through 4 were 356.4±89.6 mm3, 335.9±209.7 mm3, 371.0±104.0 mm3, 363.3±61.9 mm3, respectively, and showed no significant differences between groups (p=0.996). After 4 weeks of treatment, the mean tumor volume in Group 2 was not significantly different from that in Group 1. However, after 3 and 4 weeks of treatment, the mean tumor volumes in Groups 3 and 4 were significantly smaller than in Group 1 (p<0.05) (Fig. 3). In Group 4, which received the vaccine+imatinib combination, the mean tumor volume was the smallest among the treatment groups, and compared with the other groups, the differences were all statistically significant (p<0.05) (Fig. 3, 4). The combination therapy in Group 4 had a 0.87 combination index, indicating a synergistic suppressive effect on tumor volume compared with both monotherapies.
At the end of treatment, flow cytometry using splenocytes was performed to identify the composition of the immunocytes. To identify the activated T-cells, we used CD69-PE as the detective marker. Helper T-cells (CD4) and cytotoxic T-cells (CD8), which play significant roles in cell-mediated immunity, were analyzed. In Groups 1 through 4, the proportions of activated helper T-cells were 4.53%, 6.80%, 2.99%, and 5.80%, and the proportions of activated cytotoxic T-cells were 3.84%, 6.07%, 2.38%, and 4.95%, respectively (Fig. 5). In Groups 2 and 4, helper and cytotoxic T-cells were more activated than those in Group 1. However, in Group 3, which was treated with imatinib, helper and cytotoxic T-cells were less activated compared with Groups 1 and 2. In Group 4, which was treated with the vaccine+imatinib combination, activated helper T-cells and cytotoxic T-cells were relatively low compared with Group 2.
The MVD observed at 20 sites randomly selected in each tumor section was 178.9±14.8, 148.5±9.3, 116.4±9.5, and 69.0±7.6 in Groups 1 through 4, respectively, and the differences between the groups were statistically significant (p<0.001) (Fig. 6, 7). Additionally, the combination index was 0.43 with combination therapy. Thus, we can assume that the combination therapy in Group 4 had a synergistic effect on the inhibition of angiogenesis.
Since Coley first suggested cancer treatment using the immune system, continued efforts have been made to develop and produce an effective anticancer vaccine [16]. Typical anticancer vaccines have used tumor-associated antigen (TAA) and the tumor cells themselves for anti-tumor effects, and both mechanisms augment the immune surveillance system against cancers. Tumor-infiltrating lymphocytes (TILs) are believed to play an important role in the immunologic attack on tumors. In various solid tumors, several studies on the positive correlation between the number of TILs and favorable prognosis have shown that TILs play a central role in the immune surveillance system of the host against the tumor [17-19]. TILs are mainly produced by the cell-mediated immune reaction, and they invade and induce tumor cell death. However, because tumor cells are genetically unstable and frequently mutate, anticancer vaccines that use TAAs and the tumor cells themselves have not shown great clinical success. No successful results have been found in Phase 3 studies of melanoma, although the accumulated data regarding TAAs in melanoma are considerable. In other types of tumors, similar to melanoma, successful data have not been found [19]. As a result, targeting stromal cells while bypassing the cancer cells may be an approach to overcoming the escape mechanism of cancer cells and is an area of extensive ongoing research.
Folkman et al reported the importance of neoangiogenesis in tumor growth [20], and targeting the neovasculature of tumors as an anti-tumor approach has been attempted. Wei et al reported that endothelial cell vaccines extracted from humans and calves, when applied to several lines of mouse cancer cells, showed good preventive and treatment effects [5]. We previously reported that an endothelial cell vaccine, genetically modified to reinforce the immune reaction, showed anti-tumor effects in a bladder cancer cell line by inhibiting neoangiogenesis [8]. There was no siginificant difference in tumor growth between control and HUVECs only. However, the growth of the tumor in the group treated with anticancer vaccine was significantly suppressed compared with that in the control and HUVECs only groups. Also, MVD in the group treated with anticancer vaccine was significantly lower than in the control and HUVECs only groups. In flow cytometry analysis, activated CD4 and CD8 cells were increased in the group treated with anticancer vaccine compared the control and HUVECs only groups.
In the present study, to reinforce the immune reaction by using an endothelial cell vaccine, we additionally used recombinant GM-CSF. Generally, GM-CSF is believed to consolidate the immune reaction by stimulating Langerhans cells and dendritic cells [21]. GM-CSF can also suppress the immune reaction, depending on the dosage. Reali et al reported that the tumor-suppressive effect of an anticancer vaccine increased in nude mice when daily, repeated 20 µg recombinant GM-CSF was administered intraperitoneally for 4 days by increasing activated dendritic cells in lymph nodes and activating CD4+ T-cells and CD8+ T-cells [22]. However, other studies have reported immunological suppression with high concentrations of GM-CSF, which is caused by the emergence of myeloid suppressor cells [23]. In the present study, we sought to obtain more powerful anti-tumor effects with the vaccines through weekly intraperitoneal administration of low-dose GM-CSF. Flow cytometry analysis revealed that Group 2, which was treated with vaccine, had elevated activated helper T-cells (CD4) and cytotoxic T-cells (CD8). The combination of the anticancer vaccine and GM-CSF, which was used to assist the vaccine-induced immunological reaction, suppressed angiogenesis by augmenting the immune reaction. Additionally, we can confirm the difference from the previously reported anticancer vaccines that targeted endothelial cells [5,6] because our endothelial cell vaccine suppressed tumors by inducing the cell-mediated immune reaction.
In the present study, the endothelial cell vaccine+imatinib combination had a synergistic effect in decreasing MVD and suppressing tumor growth. Imatinib, which blocks PDGFR-α, PDGFR-β, c-kit, and bcr-abl, was the first tyrosine kinase inhibitor approved for clinical use [24]. Therefore, we can infer that both anti-angiogenesis induced by PDGFR inhibition and the alteration of the immunological environment by inhibiting bcr-abl contribute to the synergistic effect of the combination therapy. The VEGF pathway is believed to play a central role in tumor neoangiogenesis. However, recent studies have suggested that the PDGF pathway also plays a very important role in tumor angiogenesis [12,13]. In bladder cancer carcinogenesis, the significance of the PDGF pathway is not well known. However, Kassouf et al reported that PDGFR-β overexpression in a bladder cancer cell line is resistant to gefitinib [9], and Black et al suggested that PDGFR inhibition is an important treatment option, based on evidence of anti-tumor effects of PDGFR-β inhibition [25]. Moreover, imatinib has been shown to suppress the activation of T-cells and deplete lymphocytes by inhibiting bcr-abl [26,27]. In the present study, Group 3, which was treated with imatinib, exhibited a suppression of activation of helper T-cells and cytotoxic T-cells. In Group 4, which was treated with vaccine and imatinib, activated helper T-cells and cytotoxic T-cells were relatively low compared with Group 2. Despite the immunologic- suppressive effect of imatinib, the synergistic effect on anti-angiogenesis and tumor growth is supported by a previous report, in which immunomodulation caused by lymphocyte depletion strengthened the immune reaction to cancer cell-specific antigen [28]. Chemotherapy generally inhibits the mitosis of lymphocytes and suppresses immune function. However, traditional cytotoxic chemotherapy activates the immune system by various mechanisms. Wrzesinski et al reported that the depletion of lymphocytes caused by cytotoxic agents promoted the differentiation of T-cells to memory cells and strengthened the antigen-specific immune reaction in cancer cells [29]. Larmonier et al reported that imatinib significantly enhanced antitumor immune responses to dendritic cell-based immunization and their results support previous studies indicating that imatinib may improve responses to tumor-specific vaccination [30]. In the present study, we can assume that the synergistic effect of combination therapy on anti-angiogenesis was induced by augmenting a specific immune reaction in endothelial cells with the lymphocyte-suppressive action of imatinib.
In the present study, an anticancer vaccine that used genetically modified endothelial cells and GM-CSF inhibited neoangiogenesis in urinary bladder cancer via a T-cell-mediated immune reaction. The PDGFR inhibitor imatinib suppressed angiogenesis of the tumor, and the imatinib+anticancer vaccine combination had a synergistic effect on anti-angiogenesis. The method we used in the present study was not tumor-specific and may be applicable to many different types of tumors. Additionally, the inhibition of angiogenesis induced by using a different approach while using an endothelial cell vaccine to overcome immunological drawbacks differentiates this study from previous studies. Although additional research is needed, we believe that the method we used may become a basis for future chemotherapeutic regimens.
Figures and Tables
FIG. 1
Mean body weights of mice during the treatment period. After 2 weeks of treatment, the mean body weights in Groups 2, 3, and 4 were less than in Group 1 (p<0.05).
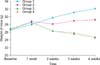
FIG. 2
Mean body weights of mice without the mass of the extracted tumor at week 4. When comparing body weights without the mass of the extracted tumor at week 4, no significant differences were observed among the treatment groups (p=0.412).
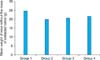
FIG. 3
Mean tumor volumes during the treatment period. At weeks 3 and 4 of the treatment period, the mean tumor volume in Groups 3 and 4 was significantly lower than in Group 1 (p<0.05). In Group 4, the mean tumor volume was the smallest among the treatment groups, and the differences were all statistically significant compared with the other groups (p<0.05). The combination index of Group 4 at week 4 was 0.87.
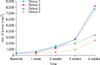
FIG. 4
Gross subcutaneous MBT-2 tumor in mice. These pictures were taken at week 4. The tumor size in Group 4 was the smallest among the treatment groups (p<0.05).
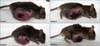
FIG. 5
Flow cytometry analysis of (A) helper T-cells (CD4+ cells) and (B) cytotoxic T-cells (CD8+ cells). The dots in the upper right quadrant represent the number of activated T-cells. More activated helper and cytotoxic T-cells were observed in Groups 2 and 4 than in Group 1. However, in Group 3, which was treated with imatinib, fewer activated helper and cytotoxic T-cells were observed compared with Groups 1 and 2. In Group 4, which was treated with vaccine and imatinib, activated helper T-cells and cytotoxic T-cells were relatively low compared with Group 2.
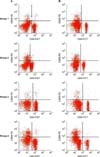
Notes
References
1. von der Maase H, Hansen SW, Roberts JT, Dogliotti L, Oliver T, Moore MJ, et al. Gemcitabine and cisplatin versus methotrexate, vinblastine, doxorubicin, and cisplatin in advanced or metastatic bladder cancer: results of a large, randomized, multinational, multicenter, phase III study. J Clin Oncol. 2000. 18:3068–3077.
2. Igawa M, Ohkuchi T, Ueki T, Ueda M, Okada K, Usui T. Usefulness and limitations of methotrexate, vinblastine, doxorubicin and cisplatin for the treatment of advanced urothelial cancer. J Urol. 1990. 144:662–665.
3. Böhle A, Brandau S. Immune mechanisms in bacillus Calmette-Guerin immunotherapy for superficial bladder cancer. J Urol. 2003. 170:964–969.
4. Dunn GP, Bruce AT, Ikeda H, Old LJ, Schreiber RD. Cancer immunoediting: from immunosurveillance to tumor escape. Nat Immunol. 2002. 3:991–998.
5. Wei YQ, Wang QR, Zhao X, Yang L, Tian L, Lu Y, et al. Immunotherapy of tumors with xenogeneic endothelial cells as a vaccine. Nat Med. 2000. 6:1160–1166.
6. Plum SM, Holaday JW, Ruiz A, Madsen JW, Fogler WE, Fortier AH. Administration of a liposomal FGF-2 peptide vaccine leads to abrogation of FGF-2-mediated angiogenesis and tumor development. Vaccine. 2000. 19:1294–1303.
7. Seavey MM, Maciag PC, Al-Rawi N, Sewell D, Paterson Y. An anti-vascular endothelial growth factor receptor 2/fetal liver kinase-1 Listeria monocytogenes anti-angiogenesis cancer vaccine for the treatment of primary and metastatic Her-2/neu+ breast tumors in a mouse model. J Immunol. 2009. 182:5537–5546.
8. Jeong H, Kwak C, Park MS, Lee SE. Novel anticancer vaccine using genetically modified endothelial cells. Korean J Urol. 2004. 45:69–76.
9. Kassouf W, Dinney CP, Brown G, McConkey DJ, Diehl AJ, Bar-Eli M, et al. Uncoupling between epidermal growth factor receptor and downstream signals defines resistance to the antiproliferative effect of Gefitinib in bladder cancer cells. Cancer Res. 2005. 65:10524–10535.
10. Yoon CY, Lee JS, Kim BS, Jeong SJ, Hong SK, Byun SS, et al. Sunitinib malate synergistically potentiates anti-tumor effect of gemcitabine in human bladder cancer cells. Korean J Urol. 2011. 52:55–63.
11. Wallerand H, Reiter RR, Ravaud A. Molecular targeting in the treatment of either advanced or metastatic bladder cancer or both according to the signalling pathways. Curr Opin Urol. 2008. 18:524–532.
12. Forsberg K, Valyi-Nagy I, Heldin CH, Herlyn M, Westermark B. Platelet-derived growth factor (PDGF) in oncogenesis: development of a vascular connective tissue stroma in xenotransplanted human melanoma producing PDGF-BB. Proc Natl Acad Sci U S A. 1993. 90:393–397.
13. Shao ZM, Nguyen M, Barsky SH. Human breast carcinoma desmoplasia is PDGF initiated. Oncogene. 2000. 19:4337–4345.
14. Gasparini G, Harris AL. Clinical importance of the determination of tumor angiogenesis in breast carcinoma: much more than a new prognostic tool. J Clin Oncol. 1995. 13:765–782.
15. Chou TC, Talalay P. Quantitative analysis of dose-effect relationships: the combined effects of multiple drugs or enzyme inhibitors. Adv Enzyme Regul. 1984. 22:27–55.
16. Coley WB. The treatment of inoperable sarcoma by bacterial toxins (the mixed toxins of the streptococcus erysipelas and the bacillus prodigiosus). Proc R Soc Med. 1910. 3:1–48.
17. Badoual C, Hans S, Rodriguez J, Peyrard S, Klein C, Agueznay Nel H, et al. Prognostic value of tumor-infiltrating CD4+ T-cell subpopulations in head and neck cancers. Clin Cancer Res. 2006. 12:465–472.
18. Ruffini E, Asioli S, Filosso PL, Lyberis P, Bruna MC, Macri L, et al. Clinical significance of tumor-infiltrating lymphocytes in lung neoplasms. Ann Thorac Surg. 2009. 87:365–371.
19. Koos D, Josephs SF, Alexandrescu DT, Chan RC, Ramos F, Bogin V, et al. Tumor vaccines in 2010: need for integration. Cell Immunol. 2010. 263:138–147.
20. Folkman J, Merler E, Abernathy C, Williams G. Isolation of a tumor factor responsible for angiogenesis. J Exp Med. 1971. 133:275–288.
21. Witmer-Pack MD, Olivier W, Valinsky J, Schuler G, Steinman RM. Granulocyte/macrophage colony-stimulating factor is essential for the viability and function of cultured murine epidermal Langerhans cells. J Exp Med. 1987. 166:1484–1498.
22. Reali E, Canter D, Zeytin H, Schlom J, Greiner JW. Comparative studies of Avipox-GM-CSF versus recombinant GM-CSF protein as immune adjuvants with different vaccine platforms. Vaccine. 2005. 23:2909–2921.
23. Serafini P, Carbley R, Noonan KA, Tan G, Bronte V, Borrello I. High-dose granulocyte-macrophage colony-stimulating factor-producing vaccines impair the immune response through the recruitment of myeloid suppressor cells. Cancer Res. 2004. 64:6337–6343.
24. Buchdunger E, Cioffi CL, Law N, Stover D, Ohno-Jones S, Druker BJ, et al. Abl protein-tyrosine kinase inhibitor STI571 inhibits in vitro signal transduction mediated by c-kit and platelet-derived growth factor receptors. J Pharmacol Exp Ther. 2000. 295:139–145.
25. Black PC, Agarwal PK, Dinney CP. Targeted therapies in bladder cancer--an update. Urol Oncol. 2007. 25:433–438.
26. Seggewiss R, Loré K, Greiner E, Magnusson MK, Price DA, Douek DC, et al. Imatinib inhibits T-cell receptor-mediated T-cell proliferation and activation in a dose-dependent manner. Blood. 2005. 105:2473–2479.
27. Dietz AB, Souan L, Knutson GJ, Bulur PA, Litzow MR, Vuk-Pavlovic S. Imatinib mesylate inhibits T-cell proliferation in vitro and delayed-type hypersensitivity in vivo. Blood. 2004. 104:1094–1099.
28. Baxevanis CN, Perez SA, Papamichail M. Combinatorial treatments including vaccines, chemotherapy and monoclonal antibodies for cancer therapy. Cancer Immunol Immunother. 2009. 58:317–324.
29. Wrzesinski C, Paulos CM, Gattinoni L, Palmer DC, Kaiser A, Yu Z, et al. Hematopoietic stem cells promote the expansion and function of adoptively transferred antitumor CD8 T cells. J Clin Invest. 2007. 117:492–501.
30. Larmonier N, Janikashvili N, LaCasse CJ, Larmonier CB, Cantrell J, Situ E, et al. Imatinib mesylate inhibits CD4+ CD25+ regulatory T cell activity and enhances active immunotherapy against BCR-ABL-tumors. J Immunol. 2008. 181:6955–6963.