Abstract
Tumor undergo uncontrolled, excessive proliferation leads to hypoxic microenvironment. To fulfill their demand for nutrient, and oxygen, tumor angiogenesis is required. Endothelial progenitor cells (EPCs) have been known to the main source of angiogenesis because of their potential to differentiation into endothelial cells. Therefore, understanding the mechanism of EPC-mediated angiogenesis in hypoxia is critical for development of cancer therapy. Recently, mitochondrial dynamics has emerged as a critical mechanism for cellular function and differentiation under hypoxic conditions. However, the role of mitochondrial dynamics in hypoxia-induced angiogenesis remains to be elucidated. In this study, we demonstrated that hypoxia-induced mitochondrial fission accelerates EPCs bioactivities. We first investigated the effect of hypoxia on EPC-mediated angiogenesis. Cell migration, invasion, and tube formation was significantly increased under hypoxic conditions; expression of EPC surface markers was unchanged. And mitochondrial fission was induced by hypoxia time-dependent manner. We found that hypoxia-induced mitochondrial fission was triggered by dynamin-related protein Drp1, specifically, phosphorylated DRP1 at Ser637, a suppression marker for mitochondrial fission, was impaired in hypoxia time-dependent manner. To confirm the role of DRP1 in EPC-mediated angiogenesis, we analyzed cell bioactivities using Mdivi-1, a selective DRP1 inhibitor, and DRP1 siRNA. DRP1 silencing or Mdivi-1 treatment dramatically reduced cell migration, invasion, and tube formation in EPCs, but the expression of EPC surface markers was unchanged. In conclusion, we uncovered a novel role of mitochondrial fission in hypoxia-induced angiogenesis. Therefore, we suggest that specific modulation of DRP1-mediated mitochondrial dynamics may be a potential therapeutic strategy in EPC-mediated tumor angiogenesis.
Cancer is one of the leading causes of death worldwide. Cancer cells undergo rapid, uncontrolled proliferation, which makes the microenvironment to hypoxic condition, results in depleted the oxygen, and insufficient blood supply. To fulfill their demands for nutrient, oxygen, and other growth factors, angiogenesis is required. Several studies have revealed that targeting angiogenesis is an effective approach for cancer therapy under hypoxic conditions [12]. However, the molecular mechanisms underlying this process remains unclear.
Endothelial progenitor cells (EPCs) were first discovered and isolated by Asahara et al. in 1997 [3]. They found that EPCs have the ability to form new blood vessels in a process known as neovascularization. EPCs can be isolated from CD34+ cells in human cord blood, peripheral blood, as well as the bone marrow. They express the surface markers for CD34, CD31, and vascular endothelial growth factor receptor-2 (VEGFR-2, KDR) [45]. It has been established that EPCs contribute to tumor development and metastasis via tumor angiogenesis [6]. In addition, they demonstrate the ability to differentiate into endothelial cells (EC), which is stimulated by pathological conditions such as hypoxia. Hypoxia-induced angiogenesis is consist of several successional steps including 1) angiogenic factors (such as VEGF, bFGF) released from hypoxic cells, and bind to EC / EPC, 2) activate MMPs to degradate the extracellular membrane (ECM), then 3) cell migration and invasion, 4) tube formation, 5) vessel stabilization, in briefly. Therefore, understanding the mechanism of hypoxia-induced angiogenesis and finding a new approach to prevent angiogenic function are essential for development of cancer therapy.
Recently, mitochondrial dynamics has emerged as a critical mechanism for cellular function in hypoxic cell signaling. Mitochondria are dynamic subcellular organelles that undergo continuous fission and fusion to maintain mitochondrial function and energy metabolism [78]. These processes are tightly regulated by specific molecules, such as dynamin-related protein Drp1 for fission, and MFN1/2 and OPA1 for fusion. Homeostasis between mitochondrial fission and fusion is important for sustaining cell survival, proliferation, and differentiation. For example, mitochondrial fission induced by CDK1/cyclinB/pDRP1 axis is essential for cell mitosis [9], and phosphorylation of DRP1 at Ser637 induced by nutrient starvation sustain the cell survival via inhibition of mitochondrial fission [10]. Kasturi et al. reported that DRP1-dependent mitochondrial fission is essential for cell differentiation [11], Luchsinger et al. showed that MFN2-induced mitochondrial fusion plays critical role to maintain stem cells [12]. It has been well-established that a shift towards mitochondrial fission occurs under hypoxic conditions [1314]. Most of the studies to date have focused on the relationship between mitochondrial fission, increased ROS production, and apoptosis [1516]. A few reports have also investigated the effect of accelerated mitochondrial fission on cell migration and invasion in breast cancer [17]. However, little is known about the effect of mitochondrial fission on endothelial cells during angiogenesis.
Therefore, the aim of this study is to clarify the role of mitochondrial fission in hypoxia-induced angiogenesis. This study demonstrated that specific inhibition of DRP1-mediated mitochondrial fission attenuated the EPC bioactivities under hypoxia. We suggest the regulation of mitochondrial dynamics maybe a novel therapeutic approach for cancer therapy.
Human EPCs were isolated as previously described [18]. All experimental procedures have been reviewed and approved by the International Review Board (IRB) of Pusan National University Hospital (IRB No.05-2017-053). In brief, mononuclear cells (MNCs) were separated from human umbilical cord blood using Ficoll-Paque PLUS (GE Healthcare, Uppsala, Sweden), according to manufacturer's instructions. MNCs were cultured with the EGM-2 Bullet Kit system (Lonza, Walkersville, MD, USA) for five days in 1% gelatin-coated plates. Cultures were supplemented with 1X penicillin streptomycin (Welgene, Korea). EPCs formed spindle-shaped colonies. To generate hypoxic conditions, cells were starved with 1% FBS in EBM-2 media for 12 h, followed by a 24-h culture period at 1% O2.
Mdivi-1 was obtained from Sigma Aldrich, and was dissolved in DMSO to yield a stock concentration of 5 mM. To assess cell viability, 1×105 EPCs were seeded in 96-well plates for 24 h. Following stabilization, EPCs were treated with serially diluted Mdivi-1 (0, 3.125, 6.25, 12.5, 25, 50, 100 µM) for 24 h. The effect of Mdivi-1 on cell viability was measured using D-Plus CCK cell viability assay kit (Dongin LS, Korea), according to manufacturer's protocol. To quantify cell viability, absorbance was measured at 450 nm with a TECAN microplate reader; results were normalized and compared with those of the untreated control.
DRP1 siRNA (siDRP1) was a kind gift from Prof. Young chan Chae (UNIST), and scramble siRNA (scRNA) was purchased from Dharmacon (USA). Cells were seeded into 1% gelatin-coated 6-well plate and induced the transfection with either siDRP1 or scRNA using Lipofectamine RNAiMAX reagent (Thermo Fisher Scientific Inc.) following manufacturer's instruction. 6 h after transfection, media was replaced with EGM-2 culture media. Further experiment was conducted 24–48 h after transfection.
Human EPCs were lysed with Pro-prep (iNtRON biotechnology, Korea). Whole cell lysates (25 µg) were separated by either 8% or 15% SDS-PAGE, after which membranes were blocked with 5% skim milk for 1 h at 25℃. Membranes were probed with specific antibodies, and proteins of interest were visualized with HRP-conjugated secondary antibodies (Thermo Fisher Scientific Inc., MA, USA) and Luminata Crescendo HRP substrates (Millipore, MA, USA). Primary antibodies against mouse anti-HIF-1A (1:1000), mouse anti-DLP1 (1:1000, all above from BD Biosciences, NJ, USA), rabbit anti-phospho-DRP1 (Ser616) (1:1000), rabbit anti-phospho-DRP1 (Ser637) (1:1000, Cell Signaling Technology, Inc., MA, USA), rabbit anti-Fis1 (1:1000), rabbit anti-OPA1 (1:1000, all above Abcam, Cambridge, UK), rabbit anti-MFN1 (1:1000), and mouse anti-beta-actin (1:2500, all above Santa Cruz Biotechnology, CA, USA) were used.
Human EPC migration assays was performed using 8 µm-pore transwell inserts (Corning Inc., MA, USA). For migration assays, 2×105/ml cells were suspended in EBM-2 medium; 100 µl (20 000 cells) cell suspension was added into the upper chamber, and 500 µl EGM-2 media was placed in the lower chamber. For invasion assays, 50000 cells were seeded in Matrigel-coated transwell inserts (BD Biosciences, NJ, USA). After 24 h of incubation under normal or hypoxic conditions (1% O2), cells were fixed with 4% paraformaldehyde for 10 min, followed by staining with 0.5% crystal violet in 25% methanol for 30 min at 25℃. Cell migration and invasion were visualized with a Zeiss Axio Imager M2 optical microscope (Zeiss, Munchi, Germany), and cell counting was conducted with the Image J software.
Matrigel growth factor reduced (GFR) Basement Membrane Matrix (100 µl) (Corning Inc., MA, USA) was placed into 96-well plates, and was incubated at 37℃ and 5% CO2 for 1 h. Then, 1×104 cells were seeded under normal or hypoxic conditions. Tube formation was observed for 6–18 h. In inhibition experiments, cells were pre-treated with 12.5 µM Mdivi-1 for 24 h prior to tube formation. Total tube length was quantified using the Image J software.
Cells were suspended in FACS buffer (1% FBS, 2 mM EDTA in PBS) and stained with specific antibodies including FITC-conjugated anti-CD34, APC-conjugated CXCR, Alexa Fluor 647-conjugated CD31, PE-conjugated KDR (all from BD Biosciences, NJ, USA), and APC-conjugated c-kit (Miltenyi Biotec, Germany). All antibodies were diluted 1:100; cells were incubated in primary antibodies for 30 min at 4℃. After cells were washed twice with cold PBS, they were resuspended and analyzed with flow cytometry.
Human EPCs were seeded at a density of 4×104 cells/ml in 1% gelatin-coated cover glass. After 24 h, untreated or Mdivi-1 treated cells were cultured in either normal or 1% O2 for 24 h. Cells were then fixed with 4% paraformaldehyde for 15 min, and were permeabilized with 0.25% PBST for 10 min at room temperature. Unspecific binding was blocked (1% BSA in PBST) for 1 h at room temperature, followed by staining with mouse anti-Tom20 antibody (1:100, Santa Cruz, CA, USA) at 4℃ overnight.
All data were presented as mean±standard error of mean (SEM). Descriptive statistics were analyzed using the GraphPad Prism 5 software (GraphPad Software Inc., La Jolla, CA). We used Student's unpaired t-tests for comparison between two groups. p<0.05 was the accepted value for statistical significance.
To confirm the effect of hypoxia on EPCs bioactivities, we performed angiogenic functional studies such as migration, invasion, and tube formation assays. Results indicated that hypoxia increase cell migration and invasion by 1.7- and 1.4-fold, respectively (Figs. 1A-D). Similarly, cells under hypoxic conditions showed greater ability to form tubes as compared to that of control, which is an indicator of endothelial cell differentiation (Figs. 1E and F). To assess whether hypoxia increases EPC population, we evaluated the expression of EPC surface markers such as CD31, KDR (VEGFR), c-kit, CXCR4, and CD34 using flow cytometry. No significant change was observed between normal and hypoxic conditions. Cells that were double positive for CXCR4, a known for migration marker, and CD34, a marker of EPC, were increased approximately by 1.7-fold under hypoxic conditions (Fig. 1G). However, other markers such as c-kit, a known for stemness, and CD31, a marker of endothelial lineage, and KDR, a one of the marker of EPC, showed slightly change. Taken together, our results showed that hypoxia increases cell migration, invasion, and tube formation, but does not have an effect on the EPC population.
Recently, it has been reported that mitochondrial dynamics is one of the essential mechanisms of hypoxic cell signaling. To verify the effect of hypoxia on the mitochondrial dynamics in EPCs, we firstly detect the mitochondrial dynamics using confocal microscopy. We observed that mitochondrial fission was induced under hypoxic condition (Fig. 2A). In addition, the expression of mitochondrial fission/fusion-related gene was changed (Fig. 2B). DRP1 is a key regulator of fission, and phosphorylation of DRP1 at Ser616 is typically used as an active form of DRP1. Dephosphorylation at Ser637 on DRP1 is also known to accelerate fission. Based on our results, the expression of pDRP1 (Ser637), an inhibition marker of fission, was significantly decreased in under hypoxic conditions at time point 24 h (Fig. 2C). Unexpectedly, pDRP1 (Ser616) was decreased during hypoxia. Fis1 is an adaptor of DRP1, which retains DRP1 in the mitochondria to accelerate fission. We observed that 24 h post-hypoxia, the expression of Fis1 was slightly increased, similar pattern to pDRP1 (Ser637). The expression of MFN1, OPA1, the markers of mitochondrial fusion, were also decreased. Taken together, we suggest that pDRP1 (Ser637)/Fis1/MFN1/OPA1 orchestrate inducing mitochondrial fission under hypoxic condition.
To confirm DRP1 is involved in hypoxia-induced mitochondrial fission, we used Mdivi-1, a known specific inhibitor of DRP1. First, we determined the optimal concentration of Mdivi-1 using the CCK-8 cell viability assay. At concentrations above 25 µM, EPCs showed cytotoxicity and reduced cell viability. Therefore, the concentration of Mdivi-1 for our 24-h incubation experiments was set at 12.5 µM (Figs. 3A and B) without cell toxicity. As expected, confocal microscopy data demonstrated that hypoxia-induced mitochondrial fission was blocked by Mdivi-1 treatment (Fig. 3C). Therefore, we confirmed that hypoxia-induced mitochondrial fission is mediated by DRP1 in EPCs.
To validate the role of DRP1-mediated mitochondrial fission in hypoxia-induced EPCs bioactivities, we assessed migration, invasion, and tube formation using DRP1 siRNA and DRP1 specific inhibitor. In the presence of Mdivi-1, hypoxia-induced cell migration was blocked by 2.8-fold change, and cell invasion also showed a trend for reduction with Mdivi-1 treatment (Figs. 4A-D). In addition, the ability for tube formation was reduced during DRP1 inhibition under hypoxic conditions (Figs. 4E and F). To confirm the role of DRP1-mediated mitochondrial fission in EPC angiogenic function, we performed the DRP1 siRNA experiments. We first confirmed that DRP1 expression was dramatically decreased up to 90% (Fig. 5A). DRP1 silencing showed decreased cell migration in 1.7-fold change, and decreased in cell invasion by 2.1-fold change under hypoxia (Figs. 5B-E), similar pattern to Mdivi-1 treatment. The ability of tube formation also decreased in DRP1 silencing cells, therefore we demonstrated that DRP1-mediated mitochondrial fission is essential step for hypoxia-induced EPCs angiogenesis. As expected, no significant changes were observed in EPC surface markers with Mdivi-1 treatment (Fig. 6). Collectively, these data indicated that DRP1 is essential in hypoxia-induced EPC migration, invasion, and tube formation, but does not play a role in expression of EPC surface markers.
In this study, we demonstrated that key role of DRP1-mediated mitochondrial fission in hypoxia-induced EPCs bioactivities; this is supported by data from previous studies. We observed that hypoxia-induced mitochondrial fission occurs in EPCs, which is correlated with evidence from previous reports in neuronal cells, cardiomyocytes, and cancer cells [171920]. In addition, phosphorylated DRP1-Ser637, a suppression marker for mitochondrial fission, was impaired in hypoxic conditions. This suggested that pDRP1-Ser637 is a key molecule that mediates hypoxia-induced mitochondrial dynamics in EPCs. Coordinately, the expression of MFN1, OPA1 also showed reduction, and Fis1 tends to slightly increase under hypoxic conditions. Finally, targeting for DRP1 using Mdivi-1, a selective DRP1 inhibitor, and DRP1 siRNA, showed dramatically impaired EPC migration, invasion, and tube formation even under hypoxic conditions. Therefore, we suggest that modulation of mitochondrial fission could be key mechanism for hypoxia-induced angiogenesis.
It is the first time to discover the role of hypoxia-induced mitochondrial fission in EPC-mediated angiogenesis. While several studies have reported that ischemia reperfusion-induced mitochondrial fission is associated with decreased ROS production and apoptosis in neuronal cells and cardiomyocyte [151621], little is known about the effect of mitochondrial dynamics on angiogenesis. As hypoxia is known to affect both angiogenesis and mitochondrial function, we examined that the effect of mitochondrial dynamics on angiogenesis [22]. In agreement with our results, Zhao et al. confirmed that DRP1 plays critical roles in cell migration and invasion in breast cancer under hypoxic condition [23]. They also observed reduced cell migration and invasion under Mdivi-1 treatment, similar pattern to our result (Fig. 4A). In endothelial cells (ECs), little is known about the relationship between mitochondrial dynamics and cellular function. Lin et al. reported that decreased DRP1 and mitochondrial fusion is involved in senescence, but not in hypoxia-induced angiogenesis [24]. They demonstrated that Mdivi-1 treatment decreases cell proliferation, oxygen consumption rate (OCR), and G2/M cell cycle arrest via autophagic flux. However, the critical regulatory site for DRP1 phosphorylation during senescence remains unclear. In addition, Kashatus et al. reported that MAPK/Erk2/pDRP1 axis is critical for tumor growth [25]. They showed that targeting DRP1 impaired tumor growth, and that phosphorylation of DRP1 at Ser616 is critical for tumor development. Therefore, these reports support our hypothesis that targeting of mitochondrial dynamics is an effective strategy that not only inhibits tumor growth, but also impairs angiogenesis at tumor sites.
In our results, pDRP1 (Ser637) seems to be a key regulatory site rather than pDRP1 (Ser616). Unexpectedly, the expression of pDRP1 (Ser616) was slightly but not significantly decreased in hypoxia. The expression of MFN1, and OPA1 tends to decrease in hypoxia. We also observed the mitochondrial fission using confocal microscopy (Fig. 2A). Taken together, pDRP1 (Ser637), Fis1, MFN1, and OPA1 coordinately induce the mitochondrial fission during hypoxic condition, except pDRP1 (Ser616). Maybe there are two possible reasons to describe the expression of pDRP1-Ser616 in hypoxic condition. First, decreased expression of pDRP1-Ser637 maybe major factor to induce mitochondrial fission rather than pDRP1-Ser616 in EPC. The response to hypoxia maybe different dependent on cell types, so pDRP1-Ser637/OPA1/MFN1 orchestrate inducing mitochondrial fission especially in EPC. Second, hypoxia-induced phosphorylation site of DRP1 is controversial, yet. Because upstream signaling of pDRP1-Ser616 and pDRP1-Ser637 are different and complex. Kim et al. reported that decreased pDRP1-Ser637 induced by AKAP121/Siah2 axis plays essential role for mitochondrial fission to adapt the hypoxic condition [26], whereas Marsboom et al. reported that HIF1A/cyclin B1/CDK1 induce DRP1 phosphorylation at Ser616, which leads to mitochondrial fission and pulmonary arterial smooth muscle proliferation [27]. Taken together, our results showed that pDRP1-Ser637, OPA1, MFN1 induce the mitochondrial fission response to hypoxic condition, especially in EPCs.
However, there is a missing gap between mitochondrial dynamics and angiogenesis. To address the missing gap, there are several possible hypotheses such as energy depletion, autophagic flux, and subcellular redistribution. According to Zhao et al., they addressed the mitochondrial fission is benefit to redistribution of mitochondrial to lamellipodia, which is essential step for cell migration [23], whereas mitochondrial fusion block that process. Another hypothesis is mitochondrial fission-induced energy depletion leads to inhibit the cell migration and invasion. Silvia et al. reported that oligomycin A, an inhibitor of mitochondrial F1F0-ATPase, inhibit the cell migration, and invasion because of lack of energy source, even under the DRP1-induced mitochondrial fission [28]. The other possible hypothesis is mitochondrial fission is involved in autophagic flux. Mdivi-1 treatment induced premature senescence and impaired angiogenic function by suppressing autophagic flux. In contrast aberrant DRP1 expression restored autophagic flux and angiogenic function [24]. To address the precise mechanism of mitochondrial fission in EPC-mediated angiogenesis, further experiment is required.
Mdivi-1, a selective DRP1 inhibitor, has been reported to be a potential therapeutic target for cancer due to its anti-cancer effect [29]. Previous studies generally used 50 µM Mdivi-1 for treatment in cancer cells (IC50=50 µM) [30]. However, the sensitivity of EPCs to Mdivi-1 was four times higher as compared with that of cancer cells (Figs. 3A and B). According to our results, a dose of 12.5 µM Mdivi-1 exerted no significant effect on EPC viability, with or without serum starvation. To minimize cell toxicity, 12.5 µM Mdivi-1 was used for further studies. We investigated the effect of Mdivi-1 on EPC bioactivities, namely, cell migration, invasion, and tube formation under hypoxic condition. Mdivi-1 treatment showed significantly decreased migration, invasion, and tube formation under hypoxia condition. That results showed similar pattern to DRP1 silencing experiment. Therefore, we suggest that targeting of DRP1 could be effective in preventing angiogenesis. However, we did not observe any significant effect of Mdivi-1 on EPC surface marker expression. While a slight change was detected, it was not sufficient to affect cell migration, invasion, and tube formation. Therefore, we confirmed that regulation of mitochondrial fission-fusion affects EPC bioactivities, but not the population of the EPC.
Taken together, we have presented a new perspective in cancer therapy, and have demonstrated that mitochondrial dynamics plays an important role in tumor angiogenesis. Furthermore, we suggest that specific modulation of mitochondrial dynamics under hypoxic conditions can be used as a potential therapeutic strategy in tumor angiogenesis.
Figures and Tables
Fig. 1
Hypoxia enhances EPC bioactivities.
(A) Human EPCs were incubated under 1% O2 hypoxic or 20% O2 for 24 h. The migration assay was performed with a Boyden chamber. Cell migration was visualized by microscopy (magnification 200×). (B) Quantification of the number of migrating cells. Values represent mean±SEM. Experiments were performed in triplicates. **p<0.01. (C) Invasion assays was conducted with or without hypoxia in EPCs. (D) Quantification of invading cells. Values represent mean±SEM. Experiments were performed in triplicates. *p<0.05. (E) Representative images of hypoxia-induced tube formation in EPCs. EPCs were cultured on Matrigel GFR under normal or hypoxic conditions for 18 h. (F) Quantification of total tube length was performed using the Image J software. Experiments were conducted in triplicates. **p<0.01, as compared with that of control. (G) EPCs surface marker expression analyzed by flow cytometry.
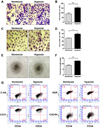
Fig. 2
Hypoxia-induced mitochondrial fission in EPCs.
(A) Human EPCs were cultured under hypoxia or normoxia for 24 h, and mitochondrial fission was observed using confocal microscopy. TOM20 was used as a mitochondrial marker, and nuclei were stained with DAPI. The white square represents magnification, as shown in the bottom panel. Scale bar=10 µm. (B) Hypoxia time-dependent mitochondrial dynamics-related gene expression was determined via immunoblot. EPCs were incubated under 1% O2 hypoxia or normoxia for the indicated time, and cells were harvested. Total DRP1, pDRP1 (S616), pDRP1 (S637), were used as mitochondrial fission markers, and OPA1, MFN1 were used as the markers of mitochondrial fusion, HIF-1A was used as a hypoxic marker, and β-actin was used for loading control. (C) Quantification of pDRP1 (S637) expression was attenuated during hypoxia. Three independent experiment were performed to statistical analysis. *p<0.05.
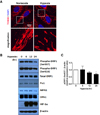
Fig. 3
DRP1-dependent mitochondrial fission in EPCs.
(A, B) EPCs were treated with Mdivi-1 for 24 h with serially diluted Mdivi-1 (0–100 µM) in full (A) or starvation (B) media. Cell viability was analyzed and quantified using the CCK-8 assay. Values were normalized to negative control. All experiments were performed in at least triplicates. **p<0.01, ***p<0.001. (C) Cells were treated with 12.5 µM Mdivi-1, and cultured at 1% O2 for 24 h. Mitochondrial morphology was observed using confocal microscopy. White square indicates magnification, as shown in bottom panels. Scale bar=10 µm. TOM20 was used for mitochondrial staining, and DAPI was used for nuclear staining.
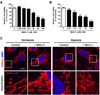
Fig. 4
Mdivi-1 inhibits EPCs bioactivities under hypoxic condition.
(A) EPCs were pre-treated with 12.5 µM Mdivi-1 for 24 h. Cells were seeded into the upper chamber with or without hypoxia for an additional 24 h. (B) Mdivi-1 pre-treated and untreated cells were seeded into Matrigel-coated transwell, and were cultured under normoxia or hypoxia for 24 h. Representative image from three independent experiments is shown. (C, D) Migrating (C) and invading cells (D) were quantified using the Image J software. All experiments were performed in at least triplicates. *p<0.05, **p<0.01. (E) EPCs were pretreated with 12.5 µM Mdivi-1 for 24 h, then seeded into Matrigel to induce tube formation under normal or hypoxic conditions. Tube structure was observed at 18 h. (F) Total tube length was measured. All experiments were performed in triplicates. *p<0.05, NS, no significant.
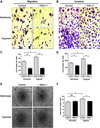
Fig. 5
DRP1 silencing inhibits hypoxia-induced angiogenic function.
(A) EPCs were transiently induced with DRP1 siRNA for 48 h. Cells were harvested and detected the expression of DRP1 to confirm silencing. DRP1 siRNA dose-dependent transduction showed decreased expression. (B) DRP1 silencing cells were seeded into the upper chamber with or without hypoxia for 6 h. DRP1 silencing cells showed decreased cell migration ability with or without hypoxic condition. (C) DRP1 silencing cells were seeded into Matrigel-coated transwell, and incubated under normoxic or hypoxic condition for 24 h. (D, E) Migrating (D) and Invading cells (E) were quantified using the Image J software. All experiment were conducted in at least triplicates. *p<0.05, **p<0.01. (F) DRP1 silencing attenuated the ability of tube formation in the hypoxic condition. Tube structure was observed at 18 h. (G) Total tube length was measured using the Image J software. All experiment were performed at least triplicates. *p<0.05.
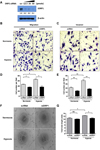
Fig. 6
Mitochondrial dynamics does not influence the expression of EPC surface markers.
The effect of Mdivi-1 on the expression of EPCs surface marker was observed using flow cytometry analysis. EPCs were cultured with or without 12.5 µM Mdivi-1 for 24 h under hypoxia or normoxia; expression of surface markers CD34 and C-kit/CD31/KDR/CXCR4 was analyzed.
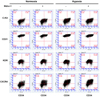
ACKNOWLEDGEMENTS
This work was supported by the National Research Foundation of Korea (NRF) grant funded by the Korea government (MSIP) (NRF-2015M3A9B4066493, NRF-2015R1A2A2A01004908, NRF-2015R1A5A2009656).
Notes
References
1. Ichihara E, Kiura K, Tanimoto M. Targeting angiogenesis in cancer therapy. Acta Med Okayama. 2011; 65:353–362.
2. Zhao Y, Adjei AA. Targeting angiogenesis in cancer therapy: moving beyond vascular endothelial growth factor. Oncologist. 2015; 20:660–673.


3. Asahara T, Murohara T, Sullivan A, Silver M, van der Zee R, Li T, Witzenbichler B, Schatteman G, Isner JM. Isolation of putative progenitor endothelial cells for angiogenesis. Science. 1997; 275:964–967.


4. Medina RJ, O'Neill CL, Sweeney M, Guduric-Fuchs J, Gardiner TA, Simpson DA, Stitt AW. Molecular analysis of endothelial progenitor cell (EPC) subtypes reveals two distinct cell populations with different identities. BMC Med Genomics. 2010; 3:18.


5. Hager G, Holnthoner W, Wolbank S, Husa AM, Godthardt K, Redl H, Gabriel C. Three specific antigens to isolate endothelial progenitor cells from human liposuction material. Cytotherapy. 2013; 15:1426–1435.


6. Marçola M, Rodrigues CE. Endothelial progenitor cells in tumor angiogenesis: another brick in the wall. Stem Cells Int. 2015; 2015:832649.


7. Ni HM, Williams JA, Ding WX. Mitochondrial dynamics and mitochondrial quality control. Redox Biol. 2015; 4:6–13.


8. Wai T, Langer T. Mitochondrial dynamics and metabolic regulation. Trends Endocrinol Metab. 2016; 27:105–117.


9. Otera H, Ishihara N, Mihara K. New insights into the function and regulation of mitochondrial fission. Biochim Biophys Acta. 2013; 1833:1256–1268.


10. Gao AW, Cantó C, Houtkooper RH. Mitochondrial response to nutrient availability and its role in metabolic disease. EMBO Mol Med. 2014; 6:580–589.


11. Mitra K, Rikhy R, Lilly M, Lippincott-Schwartz J. DRP1-dependent mitochondrial fission initiates follicle cell differentiation during Drosophila oogenesis. J Cell Biol. 2012; 197:487–497.


12. Luchsinger LL, de Almeida MJ, Corrigan DJ, Mumau M, Snoeck HW. Mitofusin 2 maintains haematopoietic stem cells with extensive lymphoid potential. Nature. 2016; 529:528–531.


13. Wolin MS. Novel role for the regulation of mitochondrial fission by hypoxia inducible factor-1α in the control of smooth muscle remodeling and progression of pulmonary hypertension. Circ Res. 2012; 110:1395–1397.


14. Wu W, Lin C, Wu K, Jiang L, Wang X, Li W, Zhuang H, Zhang X, Chen H, Li S, Yang Y, Lu Y, Wang J, Zhu R, Zhang L, Sui S, Tan N, Zhao B, Zhang J, Li L, Feng D. FUNDC1 regulates mitochondrial dynamics at the ER-mitochondrial contact site under hypoxic conditions. EMBO J. 2016; 35:1368–1384.
15. Yu T, Sheu SS, Robotham JL, Yoon Y. Mitochondrial fission mediates high glucose-induced cell death through elevated production of reactive oxygen species. Cardiovasc Res. 2008; 79:341–351.


16. Sheridan C, Martin SJ. Mitochondrial fission/fusion dynamics and apoptosis. Mitochondrion. 2010; 10:640–648.


17. Han XJ, Yang ZJ, Jiang LP, Wei YF, Liao MF, Qian Y, Li Y, Huang X, Wang JB, Xin HB, Wan YY. Mitochondrial dynamics regulates hypoxia-induced migration and antineoplastic activity of cisplatin in breast cancer cells. Int J Oncol. 2015; 46:691–700.


18. Kwon SM, Lee JH, Lee SH, Jung SY, Kim DY, Kang SH, Yoo SY, Hong JK, Park JH, Kim JH, Kim SW, Kim YJ, Lee SJ, Kim HG, Asahara T. Cross talk with hematopoietic cells regulates the endothelial progenitor cell differentiation of CD34 positive cells. PLoS One. 2014; 9:e106310.


19. Sanderson TH, Raghunayakula S, Kumar R. Neuronal hypoxia disrupts mitochondrial fusion. Neuroscience. 2015; 301:71–78.


20. Neary MT, Ng KE, Ludtmann MH, Hall AR, Piotrowska I, Ong SB, Hausenloy DJ, Mohun TJ, Abramov AY, Breckenridge RA. Hypoxia signaling controls postnatal changes in cardiac mitochondrial morphology and function. J Mol Cell Cardiol. 2014; 74:340–352.


21. Bertholet AM, Delerue T, Millet AM, Moulis MF, David C, Daloyau M, Arnauné-Pelloquin L, Davezac N, Mils V, Miquel MC, Rojo M, Belenguer P. Mitochondrial fusion/fission dynamics in neurodegeneration and neuronal plasticity. Neurobiol Dis. 2016; 90:3–19.


22. Shen T, Wang N, Yu X, Shi J, Li Q, Zhang C, Fu L, Wang S, Xing Y, Zheng X, Yu L, Zhu D. The critical role of dynamin-related protein 1 in hypoxia-induced pulmonary vascular angiogenesis. J Cell Biochem. 2015; 116:1993–2007.


23. Zhao J, Zhang J, Yu M, Xie Y, Huang Y, Wolff DW, Abel PW, Tu Y. Mitochondrial dynamics regulates migration and invasion of breast cancer cells. Oncogene. 2013; 32:4814–4824.


24. Lin JR, Shen WL, Yan C, Gao PJ. Downregulation of dynamin-related protein 1 contributes to impaired autophagic flux and angiogenic function in senescent endothelial cells. Arterioscler Thromb Vasc Biol. 2015; 35:1413–1422.


25. Kashatus JA, Nascimento A, Myers LJ, Sher A, Byrne FL, Hoehn KL, Counter CM, Kashatus DF. Erk2 phosphorylation of Drp1 promotes mitochondrial fission and MAPK-driven tumor growth. Mol Cell. 2015; 57:537–551.


26. Kim H, Scimia MC, Wilkinson D, Trelles RD, Wood MR, Bowtell D, Dillin A, Mercola M, Ronai ZA. Fine-tuning of Drp1/Fis1 availability by AKAP121/Siah2 regulates mitochondrial adaptation to hypoxia. Mol Cell. 2011; 44:532–544.


27. Marsboom G, Toth PT, Ryan JJ, Hong Z, Wu X, Fang YH, Thenappan T, Piao L, Zhang HJ, Pogoriler J, Chen Y, Morrow E, Weir EK, Rehman J, Archer SL. Dynamin-related protein 1-mediated mitochondrial mitotic fission permits hyperproliferation of vascular smooth muscle cells and offers a novel therapeutic target in pulmonary hypertension. Circ Res. 2012; 110:1484–1497.


28. Campello S, Lacalle RA, Bettella M, Mañes S, Scorrano L, Viola A. Orchestration of lymphocyte chemotaxis by mitochondrial dynamics. J Exp Med. 2006; 203:2879–2886.

