Abstract
Background
Accumulating evidence has suggested that nitric oxide (NO) is involved in the regulation of insulin sensitivity in skeletal muscle. Recent studies also suggested NO as an important molecule regulating mitochondrial biogenesis. This study examined the effect of the NO donor, 3-morpholinosydnonimine (SIN-1), on glucose metabolism in skeletal muscle and tested the hypothesis that NO's effect on glucose metabolism is mediated by its effect on mitochondrial function.
Methods
In Sprague-Dawley (SD) rats treated with SIN-1 for 4 weeks, insulin sensitivity was measured by a glucose clamp study. Triglyceride content and fatty acid oxidation were measured in the skeletal muscle. In addition, mitochondrial DNA content and mRNA expression of mitochondrial biogenesis markers were assessed by real-time polymerase chain reaction and expression of insulin receptor substrate (IRS)-1 and Akt were examined by Western blot analysis in skeletal muscle. In C2C12 cells, insulin sensitivity was measured by 2-deoxyglucose uptake and Western blot analysis was used to examine the expression of IRS-1 and Akt.
Results
SIN-1 improved insulin sensitivity in C2C12 cells and skeletal muscles of SD rats. In addition, SIN-1 decreased triglyceride content and increased fatty acid oxidation in skeletal muscle. Mitochondrial DNA contents and biogenesis in the skeletal muscle were increased by SIN-1 treatment. Moreover, SIN-1 increased the expression of phosphor-IRS-1 and phosphor-Akt in the skeletal muscle and muscle cells.
Nitric oxide (NO) is an important bioactive molecule that plays a variety of role in normal physiological as well as pathological conditions1,2). NO is synthesized by NO synthase (NOS) such as endothelial NOS (eNOS), neuronal NOS (nNOS), and inducible NOS (iNOS) and NOSs are expressed in skeletal muscle3-5). Accumulating evidence has suggested that exogenous NO stimulates glucose transport in isolated skeletal muscles6-8) and increases glucose transporter isoform 4 (GLUT4) expression in skeletal muscle cells9). In addition, it has been reported that eNOS null mice are insulin resistant10,11). These data suggest that NO might be involved in the regulation of insulin sensitivity in skeletal muscle.
Mitochondria are the major site of intracellular respiration and energy metabolism. Mitochondrial function in skeletal muscle is regarded as an important determinant of whole body insulin sensitivity12). Recently, it was reported that NO generated by eNOS stimulates mitochondrial biogenesis in skeletal muscle and muscle cells13). From these data, we hypothesized that NO improves insulin sensitivity by mediating mitochondrial biogenesis in skeletal muscle.
To examine this hypothesis, we investigated the effect of NO donor, 3-morpholinosydnonimine (SIN-1), on glucose metabolism in skeletal muscle both in vitro and in vivo. We also investigated the downstream mechanism by which NO mediates glucose metabolism.
C2C12 myoblasts were purchased from the American Type Culture Collection (Manassas, VA). Cells were maintained in DMEM (Gibco-BRL, Gaithersburg, MD) supplemented with 10% fetal bovine serum (Hyclone, Logan, UT) at 3 7℃ in a humidified atmosphere of 95% air and 5% CO2. For differentiation into myotubes, myoblasts were grown in dishes to confluence and the culture medium was then replaced with DMEM containing 2% heat-inactivated horse serum (Hyclone) for 4~6 days. Cells were treated with SIN-1 (Sigma, St. Louis, MO) as indicated in the text.
Eight-week-old male Sprague-Dawley (SD) rats (Orient, Sungnam) were housed at ambient temperature (22 ± 1℃), with 12:12-h light-dark cycles and free access to water and rat chow. SIN-1 treatment was conducted by 3 mg/kg/day for 4 weeks for all animal experiments. For muscle studies, rats were anesthetized with pentobarbital sodium (5 mg/100 g body weight, by intraperitoneal injection). Soleus muscles were rapidly excised and trimmed of fat and connective tissue. Subsequently, the soleus muscles were wrapped in aluminum foil and stored in -80℃ deep freezer until further analysis. All experiments were approved by the Institutional Animal Care and Use Committee at the Asan Institute for Life Sciences.
Glucose clamp experiments were performed as previously described14). Briefly, 12-week-old SD rats were fasted for 5 h and subjected to a euglycemic-hyperinsulinemic clamp. At the end of these euglycemic-hyperinsulinemic clamps, a bolus of 2-deoxy-D-[U-14C]glucose (2-DG) (20 µCi) was injected to determine the in vivo insulin-stimulated glucose uptake of soleus muscle.
C2C12 cells were cultured as described above and treated with SIN-1 as indicated in the text. Glucose uptake was performed as previously described15). Briefly, the cells were starved in serum-free medium for 2 h and exposed to 100 nM of insulin for 16 h to study basal and insulin-stimulated glucose uptake. Next, we added 2-DG (1 µCi per well) and quantified the incorporation rate.
Triglyceride content in soleus muscle was determined using the Sigma Triglyceride kit (GPO-Trinder) according to the manufacturer's protocol.
Measurement of fatty acid oxidation of soleus muscle in vitro was performed as described16) with slight modification. Fatty acid oxidation was measured using ~50 mg soleus muscle sample excised from rats. These samples were cut into ~5 mg pieces and placed in 5 mL tubes containing 2 mL Krebs-Ringer phosphate buffer (containing 1% BSA [wt/vol.], pH 7.4) and 0.074 MBq (2.04 GBq/mmoL) of [1-14C]oleic acid (Amersham Pharmacia Biotech, Piscataway, NJ). The content of the 5 mL tubes was then placed in a 50 mL tube containing 10 mL 1 N NaOH and a 3 × 5 cm filter paper strip to trap 14CO2. Incubation was at 37℃ for 1.5 h with oscillation (120 strokes/min), after which 600 µL of 2 N H2SO4 was injected into the 5 mL tube to release 14CO2. The 50 mL tube was then held at 55℃ for 3 h to allow transfer of 14CO2 to the NaOH in the peripheral well. The filter papers were minced and submerged in the NaOH for 1 h and then the content of peripheral well was transferred to scintillation fluid and counted.
The mitochondrial DNA (mtDNA) content was quantified by an ABI PRISM 7000 sequence detection system (Applied Biosystems, Foster City, CA) using the SYBR Green (Qiagen, Valencia, CA)17). Total DNA was extracted from muscle tissue of rats using a QIAamp DNA mini kit (Qiagen). The reactions were performed as follows: initial denaturing step at 95℃ for 10 minutes and 40 cycles of 95℃ for 15 seconds and 60??for 1 minute. The SYBR Green fluorescence was read at the end of each extension step (60℃). A melting curve (loss of fluorescence at a given temperature between 66℃ and 94℃) was analyzed to check the specificity of the PCR product. The primer sequences were: forward 5'-ACC AAT GTC ACC TCC TAC AG-3'; reverse 5'-TAG AAT GAT TAG TGG GGT GG-3'.
Messenger RNA (mRNA) expression of peroxisome proliferator-activated receptor γ coactivator 1α (PGC-1α), nuclear respirator factor-1 (NRF-1), mitochondrial transcription factor A (Tfam) in soleus muscles were quantified by RT-PCR analysis. Total RNA was isolated using Trizol (Invitrogen, Carlsbad, CA). For quantitative RT-PCR analysis, 2 µg of total RNA were reverse-transcribed with oligo (dt) using ReverseAid M-MuLV Reverse Transcriptase (Roche Diagnostics, Mannheim, Germany). Target cDNA levels were quantified by RT-PCR using the ABI PRISM 7000 sequence detection system (Applied Biosystems) utilizing SYBR green. The primer sequences used are shown in Table 1.
C2C12 cells and skeletal muscle tissue of rats were lysed in a lysis buffer. Proteins were separated by SDS-PAGE and then electrotransferred to nitrocellulose membranes. After incubating in blocking buffer, membranes were incubated with anti-IRS-1 (Santa Cruz Biotechnology, Santa Cruz, CA) and anti-phospho IRS-1 antibodies (Santa Cruz Biotechnology) and anti-Akt (Santa Cruz Biotechnology) and anti-phospho Akt antibodies (Cell Signaling, Beverly, MA), washed and incubated with horseradish peroxidase-conjugated secondary antibody (Santa Cruz Biotechnology). Band intensities were quantified by a densitometer. The membranes were re-blotted with anti-β-actin antibody (Sigma) to verify equal loading of protein in each lane.
Data are expressed as means ± standard error of the mean (SEM). Where applicable, statistical analysis was performed by two-tailed unpaired Student's t test or by one-way analysis of variance followed by post-hoc Tukey's multiple comparison test. Statistical significance was defined as P < 0.05. All analyses were performed using Statistical Package for Social Science (SPSS).
Administration of SIN-1 (3 mg/kg/day) for 4 weeks increased glucose infusion rate and 2-DG uptake in soleus muscles from SD rats compared with controls (Fig 1A & B). In accordance with these in vivo data, SIN-1 treatment (0.1 mM) for 18 h of C2C12 cells also increased insulin -stimulated 2-DG uptake, but not basal 2-DG uptake (Fig. 1C).
To examine whether SIN-1 affects the insulin signaling pathway, we measured phosphorylation of IRS-1 and Akt in skeletal muscle of SD rats and C2C12 cells by Western analysis. Administration of SIN-1 increased phosphorylation of IRS-1 and Akt in skeletal muscle of SD rats after insulin injection (Fig 4A & 4B). Similar to in vivo results, in C2C12 cells, SIN-1 treatment (0.1 mM for 18 h) increased phophorylation of IRS-1 and Akt compared with control cells in insulin-stimulated condition, but not in basal state (Fig. 4C & 4D).
We demonstrate here that exogenously administered NO donor increases insulin sensitivity by affecting mitochondrial biogenesis and insulin signaling pathway in skeletal muscle.
Mitochondria are the main site of intracellular respiration and energy metabolism. Growing evidence has suggested that NO induces mitochondrial biogenesis and improves mitochondrial function in mammalian cells13,18). In addition, mitochondrial biogenesis was shown to be defective in eNOS null mice13,18). In the present study, administration of NO donor enhanced the expression of mitochondrial biogenesis markers (PGC-1α, NRF-1, Tfam) and also increased mitochondrial DNA content in soleus muscles from SD rats. Administration of NO donor also significantly increased fatty acid oxidation and decreased triglyceride content in skeletal muscle. Taken together, these data indicate that NO stimulates mitochondrial biogenesis and improves mitochondrial function.
Under physiologic conditions, the eNOS is expressed in skeletal muscle4,5,19) and locally produces small amounts of NO. Small amounts of NO produced by eNOS are known to play a key role in the regulation of glucose homeostasis and energy production19). Indeed, defective eNOS-driven NO synthesis causes insulin resistance in eNOS null mice10) and NO donors stimulate glucose transport in isolated rat muscle preparations in vitro6,7,20). Additionally, the administration of L-NMMA, NOS inhibitor, in vivo resulted in the development of marked insulin resistance21-23) and hyperglycemia23), suggesting an important role of NO in muscle glucose metabolism. In this study, administration of SIN-1 increased insulin-stimulated glucose uptake in muscle cells. Similarly, administration of SIN-1 increased whole body insulin sensitivity and insulin-stimulated glucose uptake in skeletal muscle of SD rats. Previous reports suggested that the mechanisms of increase in insulin-stimulated glucose transport in vivo involve enhanced blood flow and enhanced glucose delivery to the muscle by NO released from the endothelium21,22,24). In this study, we observed that NO improved mitochondrial function in skeletal muscle. Taken together with previous studies suggesting mitochondria as an important player in insulin action in peripheral tissues12), these data suggest that NO-mediated improvement in mitochondrial function is responsible for improved insulin sensitivity.
NO is known to augment insulin-stimulated glucose uptake by skeletal muscle21). Insulin action is mediated through the insulin signaling cascades. Among molecules involved in insulin signaling pathway for glucose uptake, IRS-1 plays a critical role for insulin-stimulated glucose uptake through phosphorylation/activation of its downstream effectors including Akt25). In this study, we observed that treatment of SIN-1 increased mtDNA content in skeletal muscle of rats, and also increased phosphorylation of IRS-1 and Akt, both in vitro and in vivo. This is in agreement with previous studies showing that mitochondrial dysfunction in skeletal muscle abolishes insulin activation of IRS-1-PI3-kinase-Akt activity26). Additionally, it was reported that in muscle cells depleted of mitochondrial DNA, insulin-stimulated phosphorylation of IRS-1 and Akt2/PKB were drastically reduced27). Collectively, it is suggested that cellular mtDNA content modulates insulin signaling pathways, and that NO availability is an important factor that determines mtDNA content.
In summary, we demonstrated that NO increases glucose uptake in skeletal muscle both in vitro and in vivo by improving mitochondrial function and stimulating insulin signaling pathway. However, the precise mechanism that account for relationship between mitochondrial function and insulin signaling pathway has not been documented in this study. Further researches are needed to investigate precise molecular mechanism.
Figures and Tables
Fig. 1
SIN-1 improves insulin sensitivity in muscle. (A) Glucose infusion rate and (B) 2-DG uptake during hyperinsulinemic euglycemic clamp in skeletal muscle of SD rats after 4 weeks of SIN-1 (3 mg/kg/day) administration (n = 8 each). (C) 2-DG uptake in C2C12 cells after 18 h of incubation with or without SIN-1 (0.1 mM) in basal and insulin-stimulated condition. Data are expressed as mean ± SEM. In A-B, *P < 0.05 vs. control. In C, *P < 0.05 vs. without SIN-1 in basal condition and †P < 0.05 vs. without SIN-1 treatment in insulin-stimulated condition.
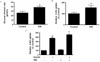
Fig. 2
SIN-1 affects fatty acid metabolism in muscle. (A) Triglyceride concentration and (B) fatty acid oxidation in skeletal muscle of SD rats after 4 weeks of SIN-1 (3 mg/kg/day) administration (n = 8 each). Data are expressed as mean ± SEM. In A-B, *P < 0.05 vs. control.
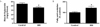
Fig. 3
SIN-1 increases mitochondrial biogenesis. The effect of SIN-1 on (A) mitochondrial DNA content and (B) mRNA expression of PGC-1α, NRF-1 and Tfam in skeletal muscle of SD rats after 4 weeks of SIN-1 (3 mg/kg/day) administration (n = 8 each). Data are expressed as mean ± SEM. In A-B, *P < 0.05 vs. control.
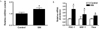
Fig. 4
SIN-1 stimulates insulin signaling pathway. (A & B) Western blot analysis showing the effect of SIN-1 on (A) IRS-1 and (B) Akt in skeletal muscle of SD rats (n = 5 each). To test the effect of SIN-1 on insulin signaling pathway, soleus muscles from SD rats were rapidly dissected after 10-min of intraperitoneal injection of insulin (regular insulin, 10 unit/kg). (C & D) Representative Western blot showing the effect of SIN-1 on (C) IRS-1 and (D) Akt in C2C12 cells after 18 h of incubation with or without SIN-1 (0.1 mM) in basal and insulin-stimulated condition. Data are expressed as mean ± SEM. In A-D, *P < 0.05 vs. without SIN-1 in basal condition (Con) and †P < 0.05 vs. without SIN-1 in insulin-stimulated condition (Con + Ins).
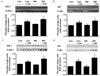
References
1. Lane P, Gross SS. Cell signaling by nitric oxide. Semin Nephrol. 1999. 19:215–229.
2. Gross SS, Wolin MS. Nitric oxide: Pathophysiological mechanisms. Annu Rev Physiol. 1995. 57:737–769.
3. Moncada S, Higgs A. The l-arginine-nitric oxide pathway. N Engl J Med. 1993. 329:2002–2012.
4. Kapur S, Bedard S, Marcotte B, Cote CH, Marette A. Expression of nitric oxide synthase in skeletal muscle: A novel role for nitric oxide as a modulator of insulin action. Diabetes. 1997. 46:1691–1700.
5. Kobzik L, Reid MB, Bredt DS, Stamler JS. Nitric oxide in skeletal muscle. Nature. 1994. 372:546–548.
6. Balon TW, Nadler JL. Evidence that nitric oxide increases glucose transport in skeletal muscle. J Appl Physiol. 1997. 82:359–363.
7. Young ME, Radda GK, Leighton B. Nitric oxide stimulates glucose transport and metabolism in rat skeletal muscle in vitro. Biochem J. 1997. 322(Pt 1):223–228.
8. Etgen GJ Jr, Fryburg DA, Gibbs EM. Nitric oxide stimulates skeletal muscle glucose transport through a calcium/contraction- and phosphatidylinositol-3-kinase-independent pathway. Diabetes. 1997. 46:1915–1919.
9. Lira VA, Soltow QA, Long JH, Betters JL, Sellman JE, Criswell DS. Nitric oxide increases GLUT4 expression and regulates AMPK signaling in skeletal muscle. Am J Physiol Endocrinol Metab. 2007. 293:E1062–E1068.
10. Duplain H, Burcelin R, Sartori C, Cook S, Egli M, Lepori M, Vollenweider P, Pedrazzini T, Nicod P, Thorens B, Scherrer U. Insulin resistance, hyperlipidemia, and hypertension in mice lacking endothelial nitric oxide synthase. Circulation. 2001. 104:342–345.
11. Shankar RR, Wu Y, Shen HQ, Zhu JS, Baron AD. Mice with gene disruption of both endothelial and neuronal nitric oxide synthase exhibit insulin resistance. Diabetes. 2000. 49:684–687.
12. Lowell BB, Shulman GI. Mitochondrial dysfunction and type 2 diabetes. Science. 2005. 307:384–387.
13. Nisoli E, Clementi E, Paolucci C, Cozzi V, Tonello C, Sciorati C, Bracale R, Valerio A, Francolini M, Moncada S, Carruba MO. Mitochondrial biogenesis in mammals: The role of endogenous nitric oxide. Science. 2003. 299:896–899.
14. Lee WJ, Song KH, Koh EH, Won JC, Kim HS, Park HS, Kim MS, Kim SW, Lee KU, Park JY. Alpha-lipoic acid increases insulin sensitivity by activating AMPK in skeletal muscle. Biochem Biophys Res Commun. 2005. 332:885–891.
15. Kase ET, Wensaas AJ, Aas V, Hojlund K, Levin K, Thoresen GH, Beck-Nielsen H, Rustan AC, Gaster M. Skeletal muscle lipid accumulation in type 2 diabetes may involve the liver x receptor pathway. Diabetes. 2005. 54:1108–1115.
16. Cha SH, Hu Z, Chohnan S, Lane MD. Inhibition of hypothalamic fatty acid synthase triggers rapid activation of fatty acid oxidation in skeletal muscle. Proc Natl Acad Sci U S A. 2005. 102:14557–14562.
17. Bogacka I, Xie H, Bray GA, Smith SR. Pioglitazone induces mitochondrial biogenesis in human subcutaneous adipose tissue in vivo. Diabetes. 2005. 54:1392–1399.
18. Nisoli E, Falcone S, Tonello C, Cozzi V, Palomba L, Fiorani M, Pisconti A, Brunelli S, Cardile A, Francolini M, Cantoni O, Carruba MO, Moncada S, Clementi E. Mitochondrial biogenesis by no yields functionally active mitochondria in mammals. Proc Natl Acad Sci U S A. 2004. 101:16507–16512.
19. Kobzik L, Stringer B, Balligand JL, Reid MB, Stamler JS. Endothelial type nitric oxide synthase in skeletal muscle fibers: Mitochondrial relationships. Biochem Biophys Res Commun. 1995. 211:375–381.
20. Higaki Y, Hirshman MF, Fujii N, Goodyear LJ. Nitric oxide increases glucose uptake through a mechanism that is distinct from the insulin and contraction pathways in rat skeletal muscle. Diabetes. 2001. 50:241–247.
21. Roy D, Perreault M, Marette A. Insulin stimulation of glucose uptake in skeletal muscles and adipose tissues in vivo is no dependent. Am J Physiol. 1998. 274:E692–E699.
22. Baron AD. The coupling of glucose metabolism and perfusion in human skeletal muscle. The potential role of endothelium-derived nitric oxide. Diabetes. 1996. 45:suppl 1. S105–S109.
23. Shankar R, Zhu JS, Ladd B, Henry D, Shen HQ, Baron AD. Central nervous system nitric oxide synthase activity regulates insulin secretion and insulin action. J Clin Invest. 1998. 102:1403–1412.
24. Baron AD, Steinberg HO, Chaker H, Leaming R, Johnson A, Brechtel G. Insulin-mediated skeletal muscle vasodilation contributes to both insulin sensitivity and responsiveness in lean humans. J Clin Invest. 1995. 96:786–792.
25. Higaki Y, Wojtaszewski JF, Hirshman MF, Withers DJ, Towery H, White MF, Goodyear LJ. Insulin receptor substrate-2 is not necessary for insulin- and exercise-stimulated glucose transport in skeletal muscle. J Biol Chem. 1999. 274:20791–20795.
26. Morino K, Petersen KF, Shulman GI. Molecular mechanisms of insulin resistance in humans and their potential links with mitochondrial dysfunction. Diabetes. 2006. 55:suppl 2. S9–S15.
27. Park SY, Lee W. The depletion of cellular mitochondrial DNA causes insulin resistance through the alteration of insulin receptor substrate-1 in rat myocytes. Diabetes Res Clin Pract. 2007. 77:suppl 1. S165–S171.