Abstract
Background and Objectives
MicroRNA 145 is known to be responsible for cellular proliferation, and its enhanced expression reportedly inhibits the retardation of vascular smooth muscle cell growth specifically. In this study, we developed a microRNA 145 nanoparticle immobilized, hyaluronic acid (HA)-coated stent.
Materials and Methods
For the gene therapy, we used disulfide cross-linked low molecular polyethylenimine as the carrier. The microRNA 145 was labeled with YOYO-1 and the fluorescent microscopy images were obtained. The release of microRNA 145 from the stent was measured with an ultra violet spectrophotometer. The downstream targeting of the c-Myc protein and green fluorescent protein was determined by Western blotting. Finally, we deployed microRNA 145/ssPEI nanoparticles immobilized on HA-coated stents in the balloon-injured external iliac artery in a rabbit restenosis model.
Results
Cellular viability of the nanoparticle-immobilized surface tested using A10 vascular smooth muscle cells showed that MSN exhibited negligible cytotoxicity. In addition, microRNA 145 and downstream signaling proteins were identified by western blots with smooth muscle cell (SMC) lysates from the transfected A10 cell, as the molecular mechanism for decreased SMC proliferation that results in the inhibition of in-stent restenosis. MicroRNA 145 released from the stent suppressed the growth of the smooth muscle at the peri-stent implantation area, resulting in the prevention of restenosis at the post-implantation. We investigated the qualitative analyses of in-stent restenosis in the rabbit model using micro-computed tomography imaging and histological staining.
One of the major treatment options for coronary heart disease is percutaneous coronary intervention, which includes percutaneous transluminal coronary angioplasty (PTCA) and coronary artery bypass graft surgery.1)2) However, PTCA results in a higher incidence of restenosis3); moreover, post-implantation, in-stent restenosis occurs in approximately 30%-50% of cases.4) Neointima formation, similar to scarring of the injured artery, eventually leads to potentially devastating effects such as a heart attack.5)6) Among the available strategies for preventing restenosis, the drug-eluting stent (DES) is widely used in clinical practice.7) The surface of the DES is loaded with drugs such as sirolimus and paclitaxel, and the DES also acts as a scaffold to hold the dilated arterial segment.7)8) However, the DES has various drawbacks, such as lacking the ability to target drug delivery to the diseased region, resulting in reduced drug effects and cytotoxicity of the drugs on the DES. Apart from these difficulties, DESs are also susceptible to "late stent thrombosis", a process or event in which the blood clots inside the stent generally occurs in ≥1 year post-stent implantation. Therefore, the present research focused on a gene therapy approach to improve stent angioplasty with gene-delivery stents.
One of the most appealing ways to prevent restenosis is by gene therapy, in which the therapeutic gene is delivered into the vascular tissue. Current procedures use either intravenous injection or a balloon catheter to introduce genes and carriers to the blood vessel. However, in cardiovascular gene therapy, we have to overcome the obstacle of delivering the therapeutic gene specifically to the target site, rather than to the blood circulatory system. One promising solution for gene therapy in-stent is the use of endovascular stents, which can act both as the gene carrier and the scaffold for localized and prolonged delivery of therapeutic genes into the diseased blood vessel wall.9)10)11)
Several reports in the literature have described gene therapy approaches using naked plasmids, viral vectors, and non-viral nano-delivery systems.12)13)14) However, several recurring issues have led researchers to reconsider their use in human clinical trials. These issues include inefficient in vivo gene transfer, high cytotoxicity, strong inflammatory reactions, and the potential risk of viral deoxyribonucleic acid (DNA) integration to the host's genome.
Disulfide cross-linked low molecular polyethylenimine (ssPEI) is a biodegradable polymer for gene delivery.15) A previous study showed the efficient transfection property of ssPEI in A10 vascular smooth muscle cells (VSMCs) line.14) We utilized microRNA 145/ssPEI nanoparticles (MSN) nanoparticles immobilized on the hyaluronic acid (HA)-coated stent to develop a safe and effective gene-eluting stent system in the treatment of restenosis. HA enhances the proliferation and migration of endothelial cells at a later stage,16) whereas microRNA 145 delivered from MSN nanoparticles can knock down the initial over-growth of VSMCs near the implanted stent.17)
MicroRNAs (miRNAs) are post-transcriptional regulators that decrease cell proliferation, survival, and metabolism. In particular, miRNA 145 (miR-145) is well-known for its potential to regulate VSMC proliferation17); hence, in our study we hypothesized that smooth muscle cell (SMC) proliferation is weakened or inhibited by the successful delivery of miR-145. In order to prove this concept, we designed a novel method to coat the stent surface with miR-145, leading to the suppression of the over-growth of SMCs.
We evaluated the microRNA 145 nanoparticle (MSN)-immobilized HA-coated stent in the New Zealand white rabbit iliac artery restenosis model. We determined its potential efficacy in the suppression of c-Myc expression and the treatment of restenosis with the New Zealand white rabbit iliac artery restenosis model. Based on these previous findings and present research, we have designed a new nano-medicine layer-by-layer (LbL)-coated stent for highly efficient delivery of therapeutic genes by targeted gene delivery.
We purchased miR-145 from Genolution Pharmaceuticals (Seoul, Korea). We transformed plasmid gWiz-luciferase (Aldevron, USA) into the competent cells, Escherichia coli DH5α, using the heat shock method. gWiz-luciferase was then propagated in bacterial cultures grown in Luria-Bertani media (Becton and Dickinson company, Franklin Lakes, NJ, USA) containing 100 µg/mL of kanamycin (Biosesang Inc., Sungnam, Korea), and purified using a mini DNA-spin kit (iNtRON Biotechnology, Seongnam, Korea). We purchased an antibody for c-Myc and green fluorescent protein (GFP) from Genolution Pharmaceuticals (Seoul, Korea). We obtained branched PEI-linked ssPEI from Pohang University of Science and Technology. We purchased HA from Lifecore Biomedical (Chaska, MN, USA). We purchased 1-Ethyl-3-(3-dimethylaminopropyl) carbodiimide from TCI (Tokyo, Japan). Finally, we purchased 3-hydroxytyramine hydrochloride and 2-(N-Morpholino) ethanesulfonic acid (MES) from Sigma Aldrich (St. Louis, MO, USA) and used them without further purification.
First, 3-hydroxytyramine hydrochloride (1400 mg), 1-Ethyl-3-(3-dimethylaminopropyl) carbodiimide (450 mg), and 5 M of hydrochloric acid (100 µL) were added to a solution of HA (1000 mg) in 0.1 M of MES (150 mL). The reaction was stirred at room temperature for 12 h. Additional 1-Ethyl-3-(3-dimethylaminopropyl) carbodiimide (450 mg) was added to the solution at each time point (3 h and 6 h). The polymer obtained was dialyzed with the MWCO 3500 membrane against 100 mM of NaCl solution for 2 days and double distilled water (DDW) for 2 days, followed by lyophilization. The conjugation rate of catechol was determined by ultraviolet-visible spectroscopy at 280 nm using dopamine standard solutions. The results showed that approximately 4.2% of the carboxylic acid groups in the HA chain were conjugated with dopamine.17) O2 plasma (10 mmHg for 10 min)-treated stents were immersed in the catechol-modified HA (HA-catechol) solution (2 mg/mL) at an acidic pH (pH<2) for 1 h and then incubated with 10 mM of Tris buffer (with a pH of 8.5) containing HA-catechol (2 mg/mL) for 10 h. After incubation, the stents were washed thoroughly with DDW and stored at 4℃ until used.
The YOYO1-labeled plasmid-miR-145 (1 µg) complexed with either ssPEI or branched polyethylenimine (bPEI) was prepared at 10 and 20 of nitrogen to phosphate (N/P) ratios for immobilization on an HA-coated stent surface. After 15 min of incubation, complexes were then immobilized on the HA-coated stent surface and incubated for 24 h at room temperature. Successful immobilization efficiency of miR-145 nanoparticles from the HA-coated stent surface was measured by fluorescent microscopy and ultra violet (UV)-spectrometer after supernatant samples were collected.
For the cell attachment study, an HA-coated stent was placed into the 24-well plate and rinsed with 70% ethanol for sterilization. After drying, the miR-145 with ssPEI nanoparticles was immobilized on the HA-coated stent surface. The A10 VSMCs were seeded on the surface of the HA-coated stent at a density of 5.0×104 cells/cm2 in Dulbecco's modified eagle medium (DMEM) medium with supplementation of antibiotics and 10% Fetal Bovine Serum (FBS). To image the tissues grown on the stent surfaces, samples were fixed with 2.5% glutaraldehyde for 2 h before they underwent serial dehydration with increasing concentrations of ethanol (40, 50, 60, 70, 80, 90, and 100%) in water for 10 min. After dehydration, the samples were dried overnight and sputter-coated using gold prior to observation under scanning electron microscopy.
The cellular viability of miR-145 nanoparticles with A10 cells was analyzed through MTS assay. miR-145 nanoparticles were immobilized on the HA-coated surface and incubated at room temperature for 24 h. After incubation, A10 cells were seeded at a density of 5×104 cells/cm2 and incubated at 37℃ in a humidified atmosphere of 5% CO2. The cells were then washed with phosphate buffered saline (PBS) and 100 µL DMEM containing 10% FBS and 1% penicillin. The viability of the cell population was analyzed using an MTS assay (Promega, Madison, WI, USA).
The A10 cells transfected with miR-145 (1 µg) were lysed after 24 h, and protein was extracted using PRO-PREP™ (iNtRON Biotechnology, Seongnam, Korea). Total protein was quantified by a bicinchoninic acid protein assay kit (Invitrogen, Waltham, MA, USA). For western blot, equal amounts of protein were separated on sodium dodecyl sulfonate-polyacrylamide gel electrophoresis, transferred onto a nitrocellulose membrane, blocked, and incubated for 1 h with anti-c-Myc and anti-GFP antibodies. After washing, the membrane was incubated with a horseradish peroxidase-labeled secondary antibody. The bands were analyzed using a luminescent image analyzer (LAS-3000, Fujifilm, Tokyo, Japan).
The animal studies were performed according to an experimental protocol approved by the Institutional Animal Care and Use Committee Ethics Committee of Chonnam National University Medical School and Chonnam National University Hospital (CNU IACUC-H-2011-5). Experiments were performed on New Zealand white rabbits (3.5 kg average weight; obtained from Damool Science, Daejeon, Korea). In vivo implantation and tissue harvesting procedures are described in the previous study.16) Briefly, the stents were implanted using fluoroscopic imaging (Philips, BV-Pulsera, Eindhoven, Netherlands) under sterile conditions. Rabbits were anesthetized with a mixture of xylazine (2.2 mg/kg) and ketamine (22 mg/kg) prior to the experiment. A heating pad was used to maintain the body temperature, and the animals received supplemental oxygen continuously via an oxygen mask. Lidocaine solution (2%) was subcutaneously administered at the cut-down site. An incision was made above the right carotid artery, and the vessel was dissected free. A Radifocus® introducer with a 4-fr introducer sheath (Terumo Co., Tokyo, Japan), pre-loaded with a guide wire, was then inserted into the vessel and advanced from the carotid artery into the distal descending aorta. The guide wire was then advanced to the proximal portion of the iliac artery. The stent was deployed to a pressure of 12 atm to achieve a stent-to-artery-size ratio range of 1.1-1.2:1.7. The bare metal stent (BMS) (3.0×9×18 mm, Co-Cr Alloy), the HA-coated stent, and MSNs/HA-immobilized stent were implanted in the left or right iliac arteries alternatively in rabbits. After the stent deployment, intravenous heparin (100 U/kg) was administered immediately. The introducer sheath was removed after successful stent deployment verified by angiography. After the procedure was completed, the rabbits were allowed to recover from anesthesia. Follow-up was performed 4 weeks post-implantation to observe sequential alteration. The animals underwent follow-up angiography in the same views as initially performed, and they were subsequently sacrificed.
The implanted stents were retrieved and stored in formaldehyde solution. The stents were placed in a V-shaped opening in clay, which had been prepared earlier in a 1.5 mL microcentrifuge tube. Stents had to be fixed firmly so that there would be no movement of the stent inside the microcentrifuge tube while imaging as performed. Omnihexol (Korea United Pharm Co., Seoul, Korea) was used as the contrast agent. A 5-mL syringe was used to inject the contrast agent (1 mL) through the opening at the center of the stents. The stents were incubated with contrast agent overnight and subjected to micro-computed tomography (CT) imaging.
The part of iliac arteries surrounding the stent was carefully removed and processed for histological analysis. The status of neointimal hyperplasia after the BMS, HA-coated stent, and MSNs/HA-immobilized stent implantations was evaluated by histological analysis. The stents obtained at day 28 post-implantation were embedded in Glycol methacrylate blocks. Sections were cut with a diamond wafer mounted by IsoMet® Low Speed Saw (Buehler, USA). The sections were stained with H&E for histological analysis. The neointimal area was measured by tracing the internal elastic lamina (IEL) and the lumen area (LA). Mean IEL and LA were calculated using an imaging analysis system. The neointima area (NA) was calculated as follows: NA=IEL-LA.
Statistical analysis was performed with the aid of commercially available software (SPSS Version 15; Chicago, IL, USA). The data were presented as mean value±standard deviation. Unpaired Student's t-test was used for the comparison of the 3 stent groups. A value of p<0.05 was considered statistically significant.
In order to visualize the immobilization of the nanoparticles over the HA-coated stent surface, the miR-145-plasmid was labeled with YOYO1 fluorescent dye. The YOYO1-labeled miR-145 was incubated with ssPEI to form the complex. The complex was then coated over the HA-coated surface. After 24 h of incubation, efficient immobilization of the complex on the HA-coated stent surface was confirmed with fluorescent microscopy (Fig. 1). The complexes were distributed uniformly over the stent, which is evident from the green color signal from the YOYO1-labelled miR-145 complexed with ssPEI. The negatively charged HA coating over the stent facilitates ionic interaction with the positively charged miR-145 nanoparticles.
The YOYO1-labeled miR-145 was used to quantitate the percentage of immobilization over the stent. The YOYO1-labeled miR-145 was incubated with ssPEI at 10 of N/P ratios to form the fluorescent miR-145 nanoparticles. After complex formation, they were immobilized over the HA-coated stent surface and placed in a plate with PBS buffer. After 24 h of incubation, the solution was collected and the absorbance was measured. The supernatant solution was transferred to UV-transparent cuvettes, and the absorbance was measured at 490 nm using a UV spectrophotometer. More than 80% of the MSNs were bound over the HA-coated surface, and the binding efficiency of the ASNs on the HA-coated surface was not affected significantly (Fig. 1).
A10 VSMC lines were used to study cell attachment on miR-145/HA-coated stents. We found that a larger number of cells were attached to the surface of the stent, indicating efficient binding of the cells to the stent surface, as illustrated in Fig. 2. Furthermore, the cells were uniformly spread over the stent, thereby aiding in the cellular uptake of the miR-145 nanoparticles as illustrated in Fig. 3. Successful immobilization and delivery of miR-145 nanoparticles to the cells attached on the stent would prevent post-angioplasty side effects such as the migration and over-growth of the VSMCs.
The plasmid luciferase was complexed with ssPEI and bPEI to test their cytotoxicity in A10 cells. An MTS assay was performed to evaluate the cytotoxicity of ssPEI with the carrier luciferase/bPEI nanoparticles as a positive control. Varying concentrations of luciferase, 1 to 3 µg, were tested for viability. The luciferase/ssPEI nanoparticles showed above 90% cell viability, when compared with the control. The viability of luciferase/bPEI was only 60% (Fig. 4).
A10 VSMCs were seeded on the miR-145 nanoparticles-immobilized and HA-coated stent surface. After 24 h of incubation, the cells were collected from the stent surface and further processed for western blotting. The total protein was isolated to evaluate the suppression of the c-Myc and GFP protein at the protein level, respectively. Western blot data indicated that c-Myc protein and GFP were reduced in miR-145/ssPEI nanoparticles-treated cells, as compared to the control carrier, miR-145/bPEI nanoparticle-treated A10 cells (Fig. 5).
Micro-CT imaging was conducted after removal of the implanted stents, to calculate the percentage of tissue deposition. A stent without HA coating was used as the control in comparison with the HA-coated stent and miR-145 nanoparticle-immobilized HA-coated stents. The in-stent restenosis (ISR) area over the stent surface was calculated according to the formula in Fig. 6. The ISR area over the bare metal stent was aggravated, as compared to the HA-coated and miR-145 nanoparticle-immobilized HA-coated stents. In particular, the ISR area over the miR-145 nanoparticle-immobilized HA-coated stent was greatly reduced due to the suppression of the overgrowth of VSMCs adhering to the stent surface, which was mediated by ribonucleic acid (RNA) interference with miR-145. When the cells attached over the miR-145 nanoparticle-immobilized HA-coated stent, the miR-145 nanoparticle complex was efficiently internalized in cells and the miR-145 expression in VSMCs was suppressed, leading to cell death (Fig. 6).
According to histopathological analysis, in-stent neointima is the interval between the internal elastic lamina and the lumen boundaries, as seen in vessel cross sections. To assess the suppressive effect of the MSN/HA-immobilized stents on restenosis post-angioplasty, the neo-intimal-to-medial layer thickness ratio was calculated and compared to the BMS and HA-coated stent-treated groups (n=4/group). Arteries were stained with H&E. Fig. 7 showed representative images from each of the groups at 4×magnification. At 4 weeks post-implantation after the injury, the lumen area after 4 weeks was 1.8±0.44, 3.0±0.15, and 4.1±0.06 for the BMS, HA-coated stent, and MSN/HA-immobilized stent groups, respectively. Pathologic areas of neointimal for the BMS, HA-coated stent, and MSN/HA-immobilized stent groups were 2.9±0.44, 0.67±0.15, and 0.37±0.06, respectively, and the differences among the groups were statistically significant (p=0.03 by analysis of variance). These results indicated that MSN/HA-immobilized stents mediated the suppression of neo-intimal hyperplasia (Fig. 7).
Cytotoxic drugs or Cytostatics that inhibit SMC growth in the lumen, are used for clinically approved restenosis inhibition therapies. Stents eluting these drugs are effective in cell proliferation inhibition,18) but they do not promote re-endothelilization of the vessel, which is crucial in the long-term treatment of restenosis.19)20)
Despite the commercial availability of DESs, their safety in translation medicine is compromised due to the drug toxicity, delayed endothelization that leads to late thrombosis, and suboptimal polymer biocompatibility. One of the proposed solutions for the problems associated with DES is the gene eluting stents. The gene eluting stents with the aid of nanotechnology could easily prevent restenosis and late thrombosis by increasing the capacity of re-endothelization.21)22)
Nanoparticles carrying genes that are coated on endovascular stents represents a novel gene delivery system, but currently available gene-coated stents lack safety and specific delivery to target cells without loss in the circulatory system. Moreover, uniform coating of stents with target genes and their effective delivery to the surrounding SMCs from the stent surface still remains a challenging task.
In terms of gene delivery carriers, viral gene carriers possess high transfection efficiency, but possess potential toxicity due to their cellular mechanism. To overcome this limitation, we focused on non-viral carriers. A cationic polymer i.e., branched PEI that forms a polyplex with nucleic acid and releases them intracellularly was used in this study.23) The higher transfection efficiency of branched PEI is similar to that of viral carriers. However, cytotoxicity was observed due to its non-degrading nature and higher charge density. For increasing the degradability, a disulfide bond that is stable in the oxidative extracellular environment, but reduced and degraded in the intracellular environment, was introduced in the PEI.24) In our study, dopamine-conjugated HA was stably coated on the stent surface, followed by the immobilization of MSNs on the HA surface of the stent. This was accomplished due to the fact that HA-linked dopamine forms a strong bond with various inorganic/organic surfaces. One of the major advantage of HA coated stent is that it promotes re-endothelization with no significant inflammation25)26) and is furthermore a natural polymer that is highly biocompatible, non-immunogenic and easily degradable. One of the major problems of DESs in the post-surgery period is the toxicity arising from the polymer still attached to the surface of the stent; however, in our system, this problem was eliminated by the use of HA as the coating material. After the release of the micro RNA 145, HA coated on the surface of the stent would promote re-endothelization and self-degrade inside the body. The results showed that microRNA 145 released from an MSN/HA immobilized stent surface was capable of reducing SMC proliferation due to the suppression of the c-Myc and other proteins in the microRNA 145 signal transduction cascades. The reduction in SMC proliferation on the MSN/HA-immobilized stent surface was confirmed in vitro, and MSN/HA-immobilized stent implantation was observed in vivo with micro CT imaging in the region near the MSN/HA-immobilized stent surface. The tissue near the stent-implanted area was subjected to histological analysis for neo-intimal hyperplasia. In our study, the enzymatic degradation of HA in the blood stream occurred slowly after a faster release of MSNs to neighboring SMCs. The mechanistic and functional studies of an MSN/HA immobilized stent are currently underway in a cardiac infarction model and will be reported elsewhere.
The micro CT imaging of the stent-implanted area showed reduced ISR rate over the MSN/HA-immobilized stent region in comparison with the HA-coated stent and BMS regions. As the restenosis rate was reduced, the neo-intimal hyperplasia formation was analyzed histologically. In the MSN/HA-immobilized stent region, suppression of neo-intimal hyperplasia was observed, whereas, in the HA-coated stent and BMS regions there was little or no suppression of neo-intimal hyperplasia. The MSN/HA-immobilized stents promoted re-endothelialization, which is the main drawback of DES usage.
In conclusion, we successfully demonstrated the coating of a biodegradable ssPEI carrier with microRNA 145 nanoparticles on the surface of HA-coated stents. Post-implantation studies were successfully in the rabbit restenosis model, and achieved the effective knock-down of the microRNA 145 and downstream protein c-Myc by microRNA 145, which retarded the undesired growth of VSMCs near the stents, thereby preventing restenosis.
Figures and Tables
Fig. 1
(A) Fluorescent microscopic image of YOYO1-labeled MSNs immobilized on the HA-coated stent surfaces. (B) The binding efficiency of YOYO1-labeled MSN on an HA-coated stent surface. YOYO1-labeled miR-145 was complexed with ssPEI at N/P ratios of 10 and 20 and was then immobilized on the HA-coated stent surface. The unbound miR-145 was measured by a UV spectrophotometer. HA: hyaluronic acid, MSN: microRNA 145/disulfide cross-linked low molecular polyethylenimine nanoparticles, ssPEI: disulfide cross-linked low molecular polyethylenimine, N/P: nitrogen to phosphate, miR: microribonucleic acid, UV: ultra violet.
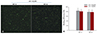
Fig. 2
SEM images of VSMCs adhering on the MSN-immobilized HA stent surface after overnight culture. SEM: scanning electron microscopy, VSMCs: vascular smooth muscle cells, MSN: microRNA 145/disulfide cross-linked low molecular polyethylenimine nanoparticles, HA: hyaluronic acid.
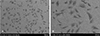
Fig. 3
The uptake of YOYO1-labeled microRNA-145/ssPEI from the HA stent was measured by confocal microscopy from 1 to 12 hours. miR: microRNA, ssPEI: disulfide cross-linked low molecular polyethylenimine, HA: hyaluronic acid.
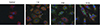
Fig. 4
The cell viability of VSMCs cultured on a luciferase/ssPEI nanoparticle-immobilized, HA stent surface. Different amounts of plasmid-luciferase (1-3 µg) were complexed with ssPEI at a fixed N/P ratio of 10 to 30 and immobilized on the HA-coated surface. The cellular viability of VSMCs on the surface was measured using MTS assay (Promega, Madison, WI, USA). VSMCs: vascular smooth muscle cells, ssPEI: disulfide cross-linked low molecular polyethylenimine, HA: hyaluronic acid, N/P: nitrogen to phosphate.
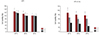
Fig. 5
Confirmation of miR-145 downstream pathway protein (c-Myc) and miR-145-GFP expression silencing after miR-145 treatments using ssPEI and bPEI carrier. Suppression of miR-145-GFP and downstream signaling proteins after the delivery of miR-145-GFP. The protein level was measured using western blotting. miR: microRNA, GFP: green fluorescent protein, ssPEI: disulfide cross-linked low molecular polyethylenimine, bPEI: branched polyethylenimine.
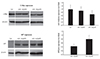
Fig. 6
Observation of post-angioplasty restenosis by micro-CT imaging. In MSN/HA-immobilized and HA-coated stents, a thin vascular overgrowth of cells was observed, and the stent linings were visible (n=3). BMS: bare metal stent, miR: microRNA, ISR: in-stent restenosis, CT: computed tomography, MSN: microRNA 145/disulfide cross-linked low molecular polyethylenimine nanoparticles HA: hyaluronic acid.
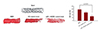
Fig. 7
Measurement of neointima by hematoxylin & eosin (H&E) staining. The iliac arteries were harvested 4 weeks after stent implantation, fixed with paraformaldehyde, embedded in Glycol methacrylate, and stained with hematoxylin & eosin (H&E) staining. The upper panel shows representative arteries from the BMS and HA-coated stent, and MSN/HA-immobilized stent groups. BMS: bare metal stent, HA: hyaluronic acid, miR: microRNA, MSN: microRNA 145/disulfide cross-linked low molecular polyethylenimine nanoparticles.
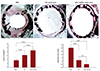
Acknowledgments
This study was supported by a research grant from the Korean Society of Cardiology (201203-7).
IKP also acknowledges the partial financial supports from the Leading Foreign Research Institute Recruitment Program, through the National Research Foundation of Korea (NRF), funded by the Ministry of Education, Science and Technology (MEST) (2011-0030034), and the Pioneer Research Center Program, through the National Research Foundation of Korea, funded by the Ministry of Science, ICT & Future Planning (2014M3C1A3053035).
References
1. Thomas MK, Gupta YK. Drug-eluting stents: a pharmacoclinical perspective. Natl Med J India. 2006; 19:195–199.
2. Zhu D, Jin X, Leng X, et al. Local gene delivery via endovascular stents coated with dodecylated chitosan-plasmid DNA nanoparticles. Int J Nanomedicine. 2010; 5:1095–1102.
3. Mehran R, Dangas G, Abizaid AS, et al. Angiographic patterns of in-stent restenosis: classification and implications for long-term outcome. Circulation. 1999; 100:1872–1878.
4. Fischman DL, Leon MB, Baim DS, et al. A randomized comparison of coronary-stent placement and balloon angioplasty in the treatment of coronary artery disease. Stent Restenosis Study Investigators. N Engl J Med. 1994; 331:496–501.
5. Babapulle MN, Eisenberg MJ. Coated stents for the prevention of restenosis: Part I. Circulation. 2002; 106:2734–2740.
6. Nikol S. Possible uses of gene therapy in reducing coronary restenosis. Heart. 1997; 78:426–428.
7. Gershlick AH. Drug eluting stents in 2005. Heart. 2005; 91(Suppl 3):iii24–iii31.
8. Morice MC, Serruys PW, Sousa JE, et al. A randomized comparison of a sirolimus-eluting stent with a standard stent for coronary revascularization. N Engl J Med. 2002; 346:1773–1780.
9. Lompré AM, Hadri L, Merlet E, et al. Efficient transduction of vascular smooth muscle cells with a translational AAV2.5 vector: A new perspective for in-stent restenosis gene therapy. Gene Ther. 2013; 20:901–912.
10. Forbes SP, Alferiev IS, Chorny M, Adamo RF, Levy RJ, Fishbein I. Modulation of NO and ROS production by AdiNOS transduced vascular cells through supplementation with L-Arg and BH4: Implications for gene therapy of restenosis. Atherosclerosis. 2013; 230:23–32.
11. Fishbein I, Chorny M, Adamo RF. Endovascular gene delivery from a stent platform: gene-eluting stents. Angiol Open Access. 2013; 1. pii:109.
12. Brito LA, Chandrasekhar S, Little SR, Amiji MM. Non-viral eNOS gene delivery and transfection with stents for the treatment of restenosis. Biomed Eng Online. 2010; 9:56.
13. Li JM, Newburger PE, Gounis MJ, Dargon P, Zhang X, Messina LM. Local arterial nanoparticle delivery of siRNA for NOX2 knockdown to prevent restenosis in an atherosclerotic rat model. Gene Ther. 2010; 17:1279–1287.
14. Che HL, Bae IH, Lim KS, et al. Suppression of post-angioplasty restenosis with an Akt1 siRNA-embedded coronary stent in a rabbit model. Biomaterials. 2012; 33:8548–8556.
15. Hwang do W, Son S, Jang J, et al. A brain-targeted rabies virus glycoprotein-disulfide linked PEI nanocarrier for delivery of neurogenic microRNA. Biomaterials. 2011; 32:4968–4975.
16. Gouëffic Y, Guilluy C, Guérin P, Patra P, Pacaud P, Loirand G. Hyaluronan induces vascular smooth muscle cell migration through RHAMM-mediated PI3K-dependent Rac activation. Cardiovasc Res. 2006; 72:339–348.
17. Muthiah M, Islam MA, Cho CS, Hwang JE, Chung IJ, Park IK. Substrate-mediated delivery of microRNA-145 through a polysorbitol-based osmotically active transporter suppresses smooth muscle cell proliferation: implications for restenosis treatment. J Biomed Nanotechnol. 2014; 10:571–579.
18. Ye YC, Xie H, Zeng Y, Zhao X, Tian Z, Zhang S. Efficacy and safety of biodegradable polymer biolimus-eluting stents versus durable polymer drug-eluting stents: a meta-analysis. PLos One. 2013; 8:e78667.
19. Al Ali J, Franck C, Filion KB, Eisenberg MJ. Efficacy and safety of drug eluting stents versus coronary artery bypass grafting: a meta-analysis of randomized controlled trials. Can J Cardiol. 2013; 29:S284.
20. Yin RX, Yang DZ, Wu JZ. Nanoparticle drug- and gene-eluting stents for the prevention and treatment of coronary restenosis. Theranostics. 2014; 4:175–200.
21. Hong YJ, Jeong MH, Ahn Y, Kang JC. The efficacy and safety of drug-eluting stents in patients with acute myocardial infarction: results from Korea Acute Myocardial Infarction (KAMIR). Int J Cardiol. 2013; 163:1–4.
22. Paul A, Shao W, Shum-Tim D, Prakash S. The attenuation of restenosis following arterial gene transfer using carbon nanotube coated stent incorporating TAT/DNAAng1+VEGF nanoparticles. Biomaterials. 2012; 33:7655–7664.
23. Lemkine GF, Demeneix BA. Polyethylenimines for in vivo gene delivery. Curr Opin Mol Ther. 2001; 3:178–182.
24. Li D, Tang X, Pulli B, et al. Theranostic nanoparticles based on bioreducible polyethylenimine-coated iron oxide for reduction-responsive gene delivery and magnetic resonance imaging. Int J Nanomedicine. 2014; 9:3347–3361.
25. Park S, Bhang SH, La WG, Seo J, Kim BS, Char K. Dual roles of hyaluronic acids in multilayer films capturing nanocarriers for drug-eluting coatings. Biomaterials. 2012; 33:5468–5477.
26. Travis JA, Hughes MG, Wong JM, Wagner WD, Geary RL. Hyaluronan enhances contraction of collagen by smooth muscle cells and adventitial fibroblasts: role of CD44 and implications for constrictive remodeling. Circ Res. 2001; 88:77–83.