Abstract
We evaluated the effects of a combined therapy of pre-blockade endogenous nitric oxide synthase (NOS) with N-nitro-L-arginine methyl ester (L-NAME) and continuous inhaled NO (iNO) on the gas exchange and hemodynamics of Escherichia coli pneumonia and sepsis in newborn piglets. Seven to ten day old ventilated newborn piglets were randomized into 5 groups: control, E. coli pneumonia control, pneumonia with iNO 10 ppm, pneumonia pre-treated with L-NAME 10 mg/kg, and pneumonia with the combined therapy of L-NAME pretreatment and iNO. E. coli pneumonia was induced via intratracheal instillation of Escherichia coli, which resulted in progressively decreased cardiac index and oxygen tension; increased pulmonary vascular resistance index (PVRI), intrapulmonary shunting, and developed septicemia at the end of 6 hr experiment. iNO ameliorated the progressive hypoxemia and intrapulmonary shunting without affecting the PVRI. Only two of 8 animals with L-NAME-pretreated pneumonia survived. Whereas when iNO was added to infected animals with L-NAME pretreatment, the progressive hypoxemia was abolished as a result of a decrease in intrapulmonary shunting without reverse of the high PVRI and systemic vascular resistance index induced by the L-NAME injection. This result suggests that a NOS blockade may be a possible supportive option for oxygenation by iNO treatment in neonatal Gram-negative bacterial pneumonia and sepsis.
Treatment with inhaled nitric oxide has been studied as an adjuvant therapy for a variety of pulmonary conditions. Because inhaled nitric oxide (NO) acts as a potent selective pulmonary vasodilator (1) that produces pulmonary vasodilatation in well-ventilated lung regions, it can potentially improve matching ventilation and perfusion (2, 3). However, up to 60% of patients with septic acute respiratory distress syndrome (ARDS) do not respond, or respond only minimally, to inhaled NO with improved oxygenation (4). Recent reports suggest that increased NO synthesis in the septic lung causes a poor responsiveness to inhaled NO (5). Attenuation of hypoxic pulmonary vasoconstriction may be another determinant of the non-response phenomenon to inhaled NO in the septic lung (6). Some studies have demonstrated that NO-dependent guanylated cyclase activity plays an important role in attenuating hypoxic vasoconstriction in the rabbit lung (7). Fischer et al. (8) reported that nitric oxide synthase inhibition restored hypoxic pulmonary vasoconstriction in sepsis. These reports suggest that improvement of oxygenation with inhaled NO may be augmented by blockade of endogenous nitric oxide synthase (NOS) in the setting of increased NO synthesis in the lung, such as pneumonia (9) or sepsis (10).
Prior studies on the effects of combined treatment, with blockade of NOS and NO inhalation, have been reported on both neonatal and adult models of experimental sepsis and endotoxemia (10-12), where recovery of the systemic blood pressure by blockade of NOS and simultaneous attenuation of pulmonary hypertension by NO inhalation were targeted. The findings show partially beneficial effects and suggest that this approach deserves further evaluation (11, 12). However, it is not known whether NOS blockade, in hemodynamically stable lung injury, is associated with improved oxygenation with NO inhalation. There are concerns that this approach might aggravate pulmonary hypertension, induced by NOS blockade, and compromise further oxygenation, especially in newborns.
We developed a newborn piglet model of Gram-negative bacterial pneumonia and sepsis by endotracheal instillation of Escherichia coli previously (13), which is thought to be appropriate to study these concerns. Because confined inflammation occurs, in the early phase of pneumonia, only in the lung, animals using this model are more hemodynamically stable in the early period of experiment than if the lung infection resulted from sepsis induced by intravenous infusion of bacteria. Gram-negative bacterial pneumonia with sepsis is a common and clinically important pulmonary disease associated with significant morbidity and mortality rates in humans including neonates. A recent study has reported that E. coli is responsible for one half of the early-onset blood stream infection (BSI) cases among neonates admitted to the neonatal intensive care unit (NICU) (14). BSI is a significant risk factor for the development of ventilator-associated pneumonia in neonates admitted in NICU (15). E. coli is the most common organism isolated from cultures of lung aspirates from neonates who are stillborn or die in the first 72 hr of life in developing countries (16). Therefore this model may be clinically relevant.
We performed this study to evaluate whether the pretreatment of nitric oxide synthase inhibitor can affect the responses to inhaled NO (iNO) treatment in gas exchange and hemodynamic profiles of newborn piglets with hemodynamically stable lung injury as a result of E. coli pneumonia and sepsis.
The protocol was approved by the Research Animal Laboratory Committee of Samsung Biomedical Research Institute, Seoul, Korea, and the procedures followed were in accord with institutional guidelines. Studies were performed on 7-10 day-old newborn piglets of mixed strain (Yorkshir, conventional breed, purchased from Paju farm, Paju, Kyungki-Do, Korea). Surgical preparation of neonatal piglets was initiated by sedation with ketamine (20 mg/kg intramuscular injection) and xylazine (2 mg/kg intramuscular injection) followed by thiopental anesthesia (5 mg/kg, intravenous injection). After local injection with lidocaine (1%), a tracheostomy was performed and the piglet was paralyzed with pancuronium (0.1 mg/kg, intravenous injection.) followed by hourly intravenous injections. Sedation was maintained with hourly doses of thiopental. The paralyzed piglet was placed on a time-cycled pressure-limited infant ventilator (Sechrist Infant Ventilator, Model IV-100V, Sechrist Industries, Anaheim, CA, U.S.A.) to attain an arterial O2 tension of 80-100 mm Hg and an arterial CO2 tension of 35-45 mm Hg. The right femoral artery was cannulated for arterial blood gas sampling and systemic arterial blood pressure monitoring. The right femoral vein was cannulated into the right atrium for infusion of ice-saline to monitor cardiac output which was calculated by the thermodilution method using the CO-set (Edwards Lifesciences, Irvine, CA, U.S.A.), and for administration of fluids and medications. A 5-Fr. Swan-Ganz catheter (Baxter Health-care Corp., Irvine, CA, U.S.A.) was inserted into the right external jugular vein and advanced into the pulmonary artery using direct-pressure and pressure wave monitoring. It was used for sampling of mixed venous blood and measurement of pulmonary arterial wedge pressures. An infusion of 0.9% saline containing 1 U of heparin/mL was provided at 1-2 mL/hr through the arterial catheter and the pulmonary arterial catheter, both of which were attached to a blood pressure transducer (Hewlett-Packard Model M1276A, MA, U.S.A.). A Hewlett Packard neonatal monitoring system (Hewlett-Packard Model M1276A) continuously monitored electrocardiogram, oxygen saturation and systemic arterial and pulmonary arterial pressure. Animals were maintained supine with the head of the bed elevated 20 degrees throughout the study. Constant body temperature was maintained between 38-39℃ using a warmed operating table and servo-controlled overhead heater (Airshields, Neonatal intensive care unit, Hatboro, PA, U.S.A.).
After surgery and stabilization, baseline measurements of arterial blood gases and hemodynamic parameters were recorded. Ventilator settings were changed to a peak inspiratory pressure (PIP) 30 cmH2O, rate 25/min, a peak end expiratory pressure (PEEP) 4 cmH2O and an inspiratory time (IT) of 0.6 sec. Animals were divided into five groups: 1) a sham operation control group (CON, n=6), 2) an E. coli pneumonia control (PCON, n=10), 3) a pneumonia and nitric oxide inhalation (PNO, n=10), 4) a N-nitro-L-arginine methyl ester (L-NAME) treated pneumonia (PNA, n=8) and 5) a L-NAME treated pneumonia followed by NO inhalation (PNANO, n=8). After baseline measurements of arterial blood gases, Escherichia coli pneumonia was induced in all animals except the CON group. Each anesthetized animal was placed in the supine position with the head elevated approximately 20 degrees and a 5-Fr. catheter was inserted through the endotracheal tube. The bacterial inoculum of Escherichia coli, EC69 strain (kind gift of Dr. Kwang Sik Kim, Johns Hopkins University, Baltimore, Maryland, U.S.A.) (17), 1×109 colony forming unit in 10 mL of 0.9% saline was instilled into the lung followed by a 10 mL bolus of air to disperse the bacteria into the distal lung. Piglets in the CON group were given 10 mL of 0.9% saline instead of the bacterial inoculum. The experimental protocols for each group are described in Fig. 1. L-NAME, a nonselective inhibitor of nitric oxide synthase was injected 30 min before bacterial instillation in the PNA and PNANO groups. Nitric oxide gas, 10 ppm inhalation, was started at 30 min after bacterial instillation and continued until the end of the experiment in the PNO and PNANO groups. The animals were maintained for 6 hr after the bacterial or saline instillations. Blood cultures were obtained, for each animal, at the end of experiment.
Heart rate (HR), mean arterial pressure (MAP, mmHg), mean pulmonary arterial pressure (PAP, mmHg), and cardiac output (mL/min) were measured by the femoral arterial and thermodilution pulmonary arterial catheters. Hemodynamic data were indexed to body weight in kilograms. The cardiac index (CI), systemic vascular resistance index (SVRI) and pulmonary vascular resistance index (PVRI) were calculated using standard formulas.
Intrapulmonary right-to-left shunting was calculated by the Fick equation (Ca-Cc/Cv-Cc). CcO2, CaO2 and CvO2 represent the oxygen content of the pulmonary capillary blood, the arterial blood and the mixed venous blood, respectively. PaO2 and PaCO2 were measured from the arterial and mixed venous blood samples taken from the femoral arterial catheter and from the pulmonary arterial catheter. Alveolar capillary O2 content (CcO2) was calculated using PAO2 (partial pressure of oxygen in the alveoli) from the alveolar gas equation with PAO2=[(BP-47)×(FiO2)]-PaCO2. Blood gases were analyzed using a blood gas analyzer (Ciba-Corning Diagnostics Corp., Medfield, MA, U.S.A.) and oxygen saturation was measured with a hemoximeter, which adjusted the oxygen saturation to the specific hemoglobin of a pig. Concentrations of lactate were measured using an YSI model 2300 dual analyzer (Yellow Springs Instrument Co., Yellow Springs, OH, U.S.A.).
Expiratory CO2 (PECO2) was measured, analyzed and standardized with a known concentration of CO2 on the expiratory side (mainstream) of the circuit's endotracheal tube connector using a CO2SMO (Novametrix Medical Systems Inc. Wallingford, CT, U.S.A.). PECO2 measurements and ABGA were simultaneously determined. The arterial end-tidal difference {P(a-E)CO2} was obtained by subtracting the PECO2 from PaCO2 of an arterial blood sample obtained during the sampling period. The dead space/tidal volume ratio (Vd/Vt ratio), as PaCO2-PECO2/PaCO2, was calculated using the Bohr-Enghoff method and was expressed as a ratio of tidal volume (18).
All measurements were recorded at baseline, 0 hr and subsequently every hour after bacterial or saline instillation through the trachea.
Normality test for each variable was done. Continuous variables with normal distribution were presented as mean±SD, which was analyzed by repeated-measures analysis of variance (ANOVA) within groups and compared by a oneway ANOVA with post hoc testing with the Bonferroni correction from each group at the same point. Variables without showing normal distribution were presented as median with range and analyzed by Kruskal-Wallis analysis of variance with the Bonferroni correction to detect the differences among the groups, where appropriate. All data analysis used the SAS software program, SAS Enterprise Guide 3.0 (SAS Institute Inc., Cary, NC, U.S.A.). p values below 0.05 were considered significant.
Prior to the study, no differences were observed among five groups of animals for body weight (CON group 4.2±0.4 kg, PCON group 4.6±0.7 kg, PNO group 4.3±0.5 kg, PNA group 4.6±0.9 kg, PNANO group 4.5±0.7 kg) and total hemoglobin concentration (CON group 11.3±1.1 g/dL, PCON group 11.6±2.4 g/dL, PNO group 11.1±1.4 g/dL, PNA group 11.8±2.5 g/dL, PNANO group 12.0±4.4 g/dL). Hemoglobin remained constant in all groups throughout the study period.
All infected animals had positive results for Escherichia coli on blood cultures from blood taken at the end of the experiment. All piglets in the CON, PCON and PNANO groups and 9/10 (90%) in PNO group survived, whereas 6/8 (75%) piglets in the PNA group died to the end of the experiment. Animals pretreated with L-NAME without NO inhalation were observed to be very unstable. They had very short survival times using this protocol. Therefore, we were unable to include the PNA group in the following data analysis.
The surgical control animals, with intratracheal instillation of saline, did not show any significant changes in heart rate, arterial blood lactate and pH, systemic and pulmonary hemodynamics or gas exchange during the entire period of experiment.
Arterial pH decreased progressively after E. coli instillation through the trachea. Nitric oxide inhalation (PNO) did not ameliorate this change in infected animals. Infected animals with L-NAME pre-treatment and iNO (PNANO) showed significantly higher lactate level at 6 hr compared to the animals of PCON and PNO groups (p<0.05) (Table 1).
Infected animals, with E. coli, did not show significant change in the mean arterial pressure (MAP) compared to the surgical control animals during the entire 6-hr period of the experiment (Fig. 2). PNANO animals showed marked and significant increase in MAP right after L-NAME injection; this continued 1 hr after bacterial instillation compared to the other groups (p<0.01). The cardiac index significantly decreased from 1 hr through 3 hr after bacterial instillation in all infected animals compared to the surgical controls (CON) (p<0.05). A greater decrease in cardiac index was observed in the PNANO animals compared to the PCON group from 0 hr to the end of the experiment (p<0.05), and when compared to the PNO group from 3 hr to 5 hr after bacterial instillation (p<0.05). SVRI increased slightly in the PCON and the PNO groups at 2 and 3 hr after bacterial instillation. However, a marked increase in the SVRI was observed in the PNANO group right after the L-NAME injection and throughout the experiment compared to the other groups (p<0.01).
Pulmonary arterial pressure (PAP) increased markedly and significantly in the PNANO animals right after L-NAME injection, until 1 hr after bacterial instillation (p<0.01); it remained higher compared to the CON group throughout the experiment (p<0.05) (Fig. 2). The other groups did not show an increase in the PAP. However when we calculated the pulmonary vascular resistance index (PVRI), there was a statistically significant increase in PVRI in all pneumonia groups (PCON, PNO, and PNANO) compared to the surgical controls for 3 hr after bacterial instillation (p<0.05). PVRI increased significantly for the PNANO group compared to the PCON and PNO right after L-NAME injection and remained high during the entire experimental period (p<0.01). NO did not decrease the PVRI to baseline in either the PNANO or the PNO animals. There were no differences in the PVRI in the PNO and the PCON animals after bacterial instillation.
PaO2 values in all groups, at the start of experiment, were in excess of 500 mmHg; the final PaO2 value was 460±28 mmHg in the surgical control animals (CON). Bacterial instillation through the trachea induced a progressive and significant decrease in the PaO2 level, which was 178±40 mmHg in the PCON animals at the end of experiment (p<0.01, by one-way repeated-measures ANOVA). NO inhalation ameliorates the progressive decrease in PaO2 in infected animals (PNO) (360±27 mmHg at the end of experiment, p<0.05 vs. PCON and CON). Pretreatment with L-NAME, before NO inhalation, (PNANO) completely prevented the progressive decrease in PaO2 (412±26 mmHg at 6 hr, p not significant by one-way repeated-measures ANOVA; p not significant vs. CON, p<0.05 vs. PCON) (Fig. 3).
PaCO2 levels were slightly increased from 25±1 mmHg at 0 hr to 31±2 mmHg at the end of the experiment in surgical controls; however, this did not reach statistical significance by one-way repeated measures ANOVA over time. Bacterial instillation through the trachea increased the PaCO2 level progressively (p<0.05, by one-way repeated-measures ANOVA), and the PaCO2 level increased from 27±1 mmHg at 0 hr to 57±7 mmHg at the end of the experiment for the PNO animals (p<0.05 vs. CON). INO did not affect the PaCO2 level in the infected animals (52±6 mmHg at 6 hr in PNO; p<0.05 vs. CON; p not significant vs. in PCON). However, the PNANO animals did not show this progressive increase in the PaCO2 level (p not significant by one-way repeated-measures ANOVA); the PaCO2 level, at the end of experiment, was not significantly different from that of the CON animals (47±5 mmHg, p not significant).
For the surgical control animals, venous admixture or percent intrapulmonary shunting did not change during the experiment (5±1% at 0 hr, 6±1% at 6 hr). However, bacterial instillation through the trachea increased the percent of intrapulmonary shunting progressively; the percent of shunting was 17±6% at the end of experiment in the PCON group (p<0.05 vs. CON). iNO prevented this progressive increase in percent of intrapulmonary shunting in the infected animals. The percent of intrapulmonary shunting decreased significantly at 5 and 6 hr during the experiment in the PNO animals, and at 3, 4, 5, 6 hr in the PNANO animals compared to the PCON group (p<0.05). No significant changes in the alveolar dead space volume (corrected Vd/Vt) were observed in all experimental groups. However a significantly decreased corrected Vd/Vt value was observed at 3 and 4 hr after bacterial instillation only in the PNANO group when compared to the PCON group (p<0.05).
We have demonstrated that L-NAME pretreatment potentiates the effect of NO inhalation, especially oxygenation in a newborn piglet model of E. coli pneumonia and sepsis. Augmented oxygenation, during iNO with pretreated L-NAME in infected animals, did not result from stabilization of circulatory instability or restoration of low blood pressure by L-NAME pre-treatment. This is because this newborn piglet model of E. coli pneumonia was not accompanied by circulatory collapse during the 6-hr study period. The study effects were associated with augmented gas exchange, complete decrease in intrapulmonary shunting and partial decrease in the relative dead space during ventilation of the infected animals as a result of combined treatment with pre-blockade of NOS and iNO, which was more pronounced when compared to infected animals treated with iNO only. In this model of pneumonia and sepsis, there was no significant changes observed in systemic and pulmonary arterial pressure during the 6-hr study period after intratracheal instillation of bacteria, however, there was a minor decrease in cardiac index, which caused a slight increase in SVRI and PVRI. INO did not affect the systemic and pulmonary hemodynamics. This is likely due to iNO not alleviating the decreased cardiac index. A decreased cardiac index might have resulted from gradual decrease in arterial pH in our study model. Further decrease in the cardiac index in the PNANO group might have resulted from aggravated systemic vasoconstriction and lactic acidosis from L-NAME pretreatment. The observed NO-related changes in oxygenation, that were seen in this piglet model with Gram-negative pneumonia and sepsis, are consistent with findings of clinical studies of acute respiratory distress syndrome, showing that inhaled NO may improve oxygenation without observed changes in pulmonary arterial pressure (19).
L-NAME pretreatment, in infected animals, was not compatible with survival and only two of 8 animals survived in that study group. This is consistent with our previous study. In that study we demonstrated that nonselective inhibition of NOS only is detrimental in the early phase of experimental neonatal sepsis (20). However, differences from this study are model used. In previous study sepsis was induced by intravenous injection of E. coli, which showed systemic hypotension (20). However in this study we used pneumonia and sepsis model without systemic hypotension, which was induced by intratracheal instillation of E. coli.
Hypoxemia during pneumonia, and other lung injury, results in large part from mismatch of perfusion and ventilation (21). Hypoxic pulmonary vasoconstriction (HPV), the normal physiological response that diverts blood flow from poorly to well-ventilated lung regions, has been shown to be impaired in patients with pneumonia, sepsis or the acute respiratory distress syndrome (22). This attenuation of HPV increases intrapulmonary shunting that results in systemic hypoxemia (8, 22, 23). Nitric oxide is known as one of the vasoactive mediators that alter pulmonary vascular tone during lung inflammation (24). Endogenous NO is produced by three different isoforms of nitric oxide synthase (NOS) that have been characterized as neuronal (NOS1), inducible (NOS2) and endothelial (NOS3) forms (25). Transcriptionally regulated NOS2-derived NO production reduces the pulmonary responsiveness to exogenous inhaled NO, alters pulmonary blood flow distribution, and impairs arterial oxygenation in endotoxin challenged mice (26).
Some possible explanations for this beneficial effect on oxygenation of L-NAME pretreatment added to NO inhalation are as follows. Previously, Fischer et al. has reported that NOS inhibition partly restores HPV in sepsis (8). In healthy animals, NOS inhibitors augment HPV (27). Very little is known, however, about the effect of NOS inhibitors on HPV in sepsis or pneumonia. Maurenbrecher et al. (6) has reported that response to inhaled NO is abolished over time and that this non-response to inhaled NO is likely to be related to the degree of V/Q mismatch. This may suggest an important role of HPV in endotoxin-induced lung injury. Recently Spohr et al. demonstrated that, in a murine model of endotoxemia, NOS2-derived NO production is critical for LPS-mediated development of impaired HPV (28).
In the pneumonia and sepsis model used in this study intrapulmonary shunting increased and arterial oxygen tension decreased progressively with time. Inhaled NO effectively decreased this V/Q mismatch, which resulted in amelioration of progressive hypoxemia. Inhaled NO added to L-NAME pretreatment, in infected animals, showed earlier and maximal decrease in intrapulmonary shunting. A previously published study by Putensen et al. (29) supports our findings. They demonstrated that in oleic acid-induced lung injury, in an adult canine model, improved ventilation-perfusion matching and gas exchange was most pronounced when NO was inhaled in the presence of systemic N-monomethyl-L-arginine (L-NMMA), a non-selective NOS inhibitor. Therefore, L-NAME pretreatment might restore HPV via inhibition of excessive production of endogenous nitric oxide, especially by NOS2, which augmented improved oxygenation with inhaled NO in our experimental model of neonatal pneumonia and sepsis.
A second plausible explanation is that L-NAME might attenuate a microvascular leak from a ventilator induced lung injury (VILI). A recent report suggested that L-NAME may attenuate ventilator-induced microvascular leaks and lipid peroxidation, and that NO may contribute to the development of VILI (30). In our experiment, all animals were ventilated with re-adjusted settings of high PIP, and high oxygen concentration right after bacterial or saline instillation. This was done to avoid significant hypoxemia that could cause additional deterioration of pulmonary hemodynamics. Therefore although the experiment duration was 6 hr, some degree of VILI, as a result of high ventilator settings, might have contributed to the lung injury especially in infected animals. L-NAME has a long plasma half-life of about 22 hr (31). In our pneumonia model, L-NAME pretreatment added to iNO, ameliorated hypercapnea and partly decreased the ratio of dead space to tidal volume. Therefore, there is a possibility that NOS blockade might blunt VILI during the study period, and potentiate the effect of inhaled NO in this model.
In summary, intratracheal E. coli instillation in newborn piglets resulted in progressive hypoxemia and hypercapnea with relatively minor changes in pulmonary and systemic hemodynamics. In this model of experimental neonatal Gram-negative bacterial pneumonia and sepsis, inhaled NO attenuated progressive hypoxemia via decrease in intrapulmonary shunting, but did not affect the increase in PVRI. Prior nonspecific NOS blockade, with L-NAME and treatment with exogenous iNO, resulted in resolution of progressive hypoxemia in this pneumonia and sepsis model and improved hypercapnea partially, although it was associated with aggravated elevation of PVRI and SVRI. Extrapolation of these findings to the clinical setting, suggest that iNO may have a limited therapeutic role in acute lung injury associated with neonatal Gram-negative bacterial pneumonia and sepsis. Endogenous NOS blockade may be a possible option for enhancement of inhaled NO, improving gas exchange in acute lung injury associated with neonatal Gram-negative bacterial pneumonia and sepsis.
Figures and Tables
Fig. 1
Scheme of the experimental protocol. L-NAME, administration of N-nitro-L-arginine methyl ester; E.coli/Saline, intratracheal instillation of Escherichia coli or Saline; iNO, administration of inhaled nitric oxide; Ventilator re-adjustment, Ventilator settings were changed to peak inspiratory pressure (PIP) 30 cmH2O, rate 25/min, peak end expiratory pressure (PEEP) 4 cmH2O, inspiratory time (IT) 0.6 sec. Animals in PNA were not included for the results because only two of eight survived. Measurement of variables was recorded hourly.
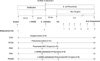
Fig. 2
Mean systemic arterial pressure (MAP), mean pulmonary arterial pressure (PAP), mean systemic vascular resistance index (SVRI), mean pulmonary vascular resistance index (PVRI) and cardiac index in the newborn piglets of 4 groups at -1, 0, 3, and 6 hr of experiment.
CON: control group; PCON: E. coli pneumonia control; PNO: E. coli pneumonia with 10 ppm nitric oxide inhalation; PNANO: E. coli pneumonia with added nitric oxide inhalation to L-NAME pretreatment (10 mg/kg). Data represent mean±standard deviation. *p<0.05 compared to CON, †p<0.05 compared to PCON.
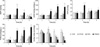
Fig. 3
Arterial oxygen tension, carbon dioxide tension, intrapulmonary shunt fraction (venous admixture %) and Vd/Vt (dead space to tidal volume ratio) in the newborn piglets of 4 groups at 0, 3, and 6 hr of experiment.
CON: control group; PCON: E. coli pneumonia control; PNO: E. coli pneumonia with 10 ppm nitric oxide inhalation; PNANO: E. coli pneumonia with added nitric oxide inhalation to L-NAME pretreatment (10 mg/kg). Data represent mean±standard deviation. *p<0.05 compared to CON, †p<0.05 compared to PCON, ‡p<0.05 compared to PNO.
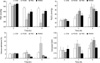
Notes
This study was supported by the Korea Research Foundation (KRF-2000-041-F00195) and intramural grant of Samsung Biomedical Research Institute (C-A4-108-1). Some of the data included here were published previously (Fig. 1, 2 of the article of Korean J Pediatr, 2003: 46: 777-83), and we got the permission formally from the board of Korean J Pediatr for the use and inclusion of that data in this article.
References
1. Roberts JD Jr, Fineman JR, Morin FC 3rd, Shaul PW, Rimar S, Schreiber MD, Polin RA, Zwass MS, Zayek MM, Gross I, Heymann MA, Zapol WM. The Inhaled Nitric Oxide Study Group. Inhaled nitric oxide and persistent pulmonary hypertension of the newborn. N Engl J Med. 1997. 336:605–610.


2. Skimming JW, DeMarco VG, Cassin S. The effects of nitric oxide inhalation on the pulmonary circulation of preterm lambs. Pediatr Res. 1995. 37:35–40.


3. Rossaint R, Falke KJ, Lopez F, Slama K, Pison U, Zapol WM. Inhaled nitric oxide for the adult respiratory distress syndrome. N Engl J Med. 1993. 328:399–405.


4. Krafft P, Fridrich P, Fitzgerald RD, Koc D, Steltzer H. Effectiveness of nitric oxide inhalation in septic ARDS. Chest. 1996. 109:486–493.


5. Holzmann A, Manktelow C, Taut FJ, Bloch KD, Zapol WM. Inhibition of nitric oxide synthase prevents hyporesponsiveness to inhaled nitric oxide in lungs from endotoxin-challenged rats. Anesthesiology. 1999. 91:215–221.


6. Maurenbrecher H, Lamy M, Deby-Dupont G, Frascarolo P, Hedenstierna G. An animal model of response and nonresponse to inhaled nitric oxide in endotoxin-induced lung injury. Chest. 2001. 120:573–581.


7. Weissmann N, Voswinckel R, Tadic A, Hardebusch T, Ghofrani HA, Schermuly RT, Seeger W, Grimminger F. Nitric oxide (NO)-dependent but not NO-independent guanylate cyclase activation attenuates hypoxic vasoconstriction in rabbit lungs. Am J Respir Cell Mol Biol. 2000. 23:222–227.


8. Fischer SR, Deyo DJ, Bone HG, McGuire R, Traber LD, Traber DL. Nitric oxide synthase inhibition restores hypoxic pulmonary vasoconstriction in sepsis. Am J Respir Crit Care Med. 1997. 156:833–839.


9. Quezado ZM, Natanson C, Karzai W, Danner RL, Koev CA, Fitz Y, Dolan DP, Richmond S, Banks SM, Wilson L, Eichacker PQ. Cardiopulmonary effects of inhaled nitric oxide in normal dogs and during E. coli pneumonia and sepsis. J Appl Physiol. 1998. 84:107–115.


10. Barrington KJ, Etches PC, Schulz R, Talbot JA, Graham AJ, Pearson RJ, Cheung PY. The hemodynamic effects of inhaled nitric oxide and endogenous nitric oxide synthesis blockade in newborn piglets during infusion of heat-killed group B streptococci. Crit Care Med. 2000. 28:800–808.


11. Pedoto A, Tassiopoulos AK, Oler A, McGraw DJ, Hoffmann SP, Camporesi EM, Hakim TS. Treatment of septic shock in rats with nitric oxide synthase inhibitors and inhaled nitric oxide. Crit Care Med. 1998. 26:2021–2028.


12. Suzuki S, Togari H, Yamaguchi N, Haas KM. Nitric oxide inhalation and nitric oxide synthase inhibitor supplement for endotoxin-induced hypotension. Pediatr Int. 2001. 43:343–349.


13. Chang YS, Ko SY, Park WS. Effect of inhaled nitric oxide on hemodynamics, gas exchange, and pulmonary inflammation in newborn piglets with Escherichia coli induced septic lungs. Korean J Pediatr Soc. 2003. 46:777–783.
14. Cordero L, Rau R, Taylor D, Ayers LW. Enteric gram-negative bacilli bloodstream infections: 17 years' experience in a neonatal intensive care unit. Am J Infect Control. 2004. 32:189–195.


15. Cordero L, Sananes M, Coley B, Hogan M, Gelman M, Ayers LW. Ventilator-associated pneumonia in very low-birth-weight infants at the time of nosocomial bloodstream infection and during airway colonization with Pseudomonas aeruginosa. Am J Infect Control. 2000. 28:333–339.


16. Park WS, Chang YS, Ko SY, Kang MJ, Han JM, Lee M. Efficacy of anti-tumor necrosis factor-alpha antibody as an adjunctive therapy in experimental Escherichia coli meningitis in the newborn piglet. Biol Neonate. 1999. 75:377–387.
17. Duke T. Neonatal pneumonia in developing countries. Arch Dis Child Fetal Neonatal Ed. 2005. 90:211–219.


18. Arnold JH, Thompson JE, Benjamin PK. Respiratory deadspace measurements in neonates during extracorporeal membrane oxygenation. Crit Care Med. 1993. 21:1895–1900.


19. Gerlach H, Rossaint R, Pappert D, Falke KJ. Time-course and dose-response of nitric oxide inhalation for systemic oxygenation and pulmonary hypertension in patients with adult respiratory distress syndrome. Eur J Clin Invest. 1993. 23:499–502.


20. Kim SS, Hwang JH, Choi CW, Shim JW, Chang YS, Park WS, Oh CK. Detrimental effects of N(omega) nitro-L-arginine methyl ester (L-NAME) in experimental Escherichia coli sepsis in the newborn piglet. J Korean Med Sci. 2003. 18:637–640.
21. Light RB, Mink SN, Wood LD. Pathophysiology of gas exchange and pulmonary perfusion in pneumococcal lobar pneumonia in dogs. J Appl Physiol. 1981. 50:524–530.


22. Marshall BE, Hanson CW, Frasch F, Marshall C. Role of hypoxic pulmonary vasoconstriction in pulmonary gas exchange and blood flow distribution. 2. Pathophysiology. Intensive Care Med. 1994. 20:379–389.
23. Ogasawara H, Koizumi T, Yamamoto H, Kubo K. Effects of a selective nitric oxide synthase inhibitor on endotoxin-induced alteration in hypoxic pulmonary vasoconstriction in sheep. J Cardiovasc Pharmacol. 2003. 42:521–526.


24. Barnes PJ, Liu SF. Regulation of pulmonary vascular tone. Pharmacol Rev. 1995. 47:87–131.
25. Moncada S, Palmer RM, Higgs EA. Nitric oxide: physiology, pathophysiology, and pharmacology. Pharmacol Rev. 1991. 43:109–142.
26. Ullrich R, Bloch KD, Ichinose F, Steudel W, Zapol WM. Hypoxic pulmonary blood flow redistribution and arterial oxygenation in endotoxin-challenged NOS2-deficient mice. J Clin Invest. 1999. 104(10):1421–1429.


27. Sprague RS, Thiemermann C, Vane JR. Endogenous endothelium-derived relaxing factor opposes hypoxic pulmonary vasoconstriction and supports blood flow to hypoxic alveoli in anesthetized rabbits. Proc Natl Acad Sci USA. 1992. 89:8711–8715.


28. Spohr F, Cornelissen AJ, Busch C, Gebhard MM, Motsch J, Martin EO, Weimann J. Role of endogenous nitric oxide (NO) in endotoxin-induced alteration of hypoxic pulmonary vasoconstriction in mice. Am J Physiol Heart Circ Physiol. 2005. 289:H823–H831.
29. Putensen C, Rasanen J, Downs JB. Effect of endogenous and inhaled nitric oxide on the ventilation-perfusion relationships in oleic-acid lung injury. Am J Respir Crit Care Med. 1994. 150:330–336.


30. Broccard AF, Feihl F, Vannay C, Markert M, Hotchkiss J, Schaller MD. Effects of L-NAME and inhaled nitric oxide on ventilator-induced lung injury in isolated, perfused rabbit lungs. Crit Care Med. 2004. 32:1872–1878.


31. Avontuur JA, Buijk SL, Bruining HA. Distribution and metabolism of N-nitro-L-arginine methyl ester in patients with septic shock. Eur J Clin Pharmacol. 1998. 54:627–631.