Abstract
Type 2 diabetes mellitus (T2DM) is characterized by hyperglycemia and dyslipidemia. Carvacrol (CAR) has demonstrated the potential to mitigate dyslipidemia. This study aims to investigate whether CAR can modulate blood glucose and lipid levels in a T2DM rat model by regulating short-chain fatty acids (SCFAs) and the GPR41/43 pathway. The T2DM rat model was induced by a high-fat diet combined with low-dose streptozocin injection and treated with oral CAR and/or mixed antibiotics. Fasting blood glucose, oral glucose tolerance, and insulin tolerance tests were assessed. Serum lipid parameters, hepatic and renal function indicators, tissue morphology, and SCFAs were measured. In vitro, high glucose (HG)-induced IEC-6 cells were treated with CAR, and optimal CAR concentration was determined. HG-induced IEC-6 cells were treated with SCFAs or/and GPR41/43 agonists. CAR significantly reduced blood lipid and glucose levels, improved tissue damage, and increased SCFA levels in feces and GPR41/43 expression in colonic tissues of T2DM rats. CAR also attenuated HG-induced apoptosis of IEC-6 cells and enhanced GPR41/43 expression. Overall, these findings suggest that CAR alleviates blood lipid and glucose abnormalities in T2DM rats by modulating SCFAs and the GPR41/43 pathway.
Type 2 diabetes mellitus (T2DM) is a prevalent metabolic disorder characterized by insulin resistance and reduced β-cell insulin secretion [1]. Its multifactorial etiology involves genetic predisposition, environmental factors, and disrupted insulin dynamics, leading to an increasing global burden of T2DM [2]. With an estimated 536.6 million individuals aged 20 to 79 affected by diabetes in 2021, this number is projected to reach 783.2 million by 2045 [3]. While current therapies encompass non-pharmacological approaches and pharmacotherapy, the quest for innovative strategies to manage T2DM remains imperative [4].
The intricate interplay between the intestinal microbiota and the host shapes a dynamic microbial ecosystem. Intestinal flora interacts with host metabolites, mucosal function, and immune system, thereby significantly influencing overall health. Mounting evidence suggests a profound connection between the intestinal microbiota and diabetes [5]. Altered intestinal barrier integrity and enhanced intestinal permeability are observed in response to various diseases and factors [6-8]. Short-chain fatty acids (SCFAs) are vital products of dietary fiber fermentation by gut bacteria [9]. They play a pivotal role in preserving gut and metabolic health [10]. Perturbations in the diversity of gut microbiota, a hallmark of diabetes, offers new avenues for therapeutic exploration [11]. Notably, SCFA levels, modulated by the abundance of SCFA-producing bacteria, can impact T2DM progression [12,13]. SCFAs exert their effects through G-protein coupled receptors (GPRs), including GPR41 and GPR43, regulating immune functions and metabolic signaling [14].
Carvacrol (CAR), a phenolic monoterpenoid present in various plant essential oils, holds promise for its diverse therapeutic properties, including diabetes prevention, anti-obesity effects, and antimicrobial activities [15]. CAR has been shown to alleviate vascular inflammation and endothelial dysfunction in diabetic animal models [16]. In T2DM rodents, CAR administration has demonstrated reductions in serum glucose and total cholesterol (TC) levels [17]. Moreover, a phytogenic supplement containing CAR has been linked to alterations in intestinal flora composition [18]. However, the precise mechanisms underlying CAR's effects remain elusive. Given the above information, it is hypothesized that CAR's regulation of intestinal flora could contribute to its beneficial impact on glucose and lipid metabolism. Thus, this study aims to elucidate whether CAR's modulation of SCFAs and the GPR41/43 pathway contributes to its ability to lower blood lipid and glucose levels in T2DM rats.
Approval for animal experimentation was obtained from the Ethics Committee of Heji Hospital Affiliated with Changzhi Medical College (DW2022003). All procedures involving animals were conducted in accordance with established guidelines, and measures were taken to minimize animal discomfort.
Male specific pathogen-free (SPF) Sprague-Dawley rats (8 weeks old, weighing 185 ± 10 g) were sourced from the Experimental Animal Center of Changzhi Medical College. Rats were housed under SPF conditions with a temperature of 22°C ± 2°C, humidity of 50% ± 10%, a 12/12 light/dark cycle, and free access to standard rat chow and water.
The T2DM rat model was established through an 8-week regimen of high-fat diet combined with low-dose streptozocin (STZ) injection [19]. Following a week of acclimation, six rats were randomly selected and fed a normal diet, constituting the normal group. The remaining 22 rats were subjected to an 8-week high-fat diet (composed of 60% corn starch, 20% casein, and 20% soybean oil) and intraperitoneally administered with 30 mg/kg STZ (Sigma-Aldrich) to induce T2DM. Fasting blood glucose (FBG) measurements were taken 72 h after STZ injection. Successful T2DM model establishment was defined as a second FBG measurement ≥ 11.1 mM. Eighteen rats were successfully induced with T2DM (success rate: 81.82%). After model establishment, the 18 T2DM rats were divided into three groups (n = 6 rat/group): T2DM group, CAR group, and CAR + mixed antibiotics (MA) group. The CAR group received daily oral gavage of 50 mg/kg CAR (282197-50G; Sigma-Aldrich) for six consecutive weeks. The normal and T2DM groups were administered equal volumes of distilled water (10 ml/kg) daily for six consecutive weeks. The CAR + MA group received 50 mg/kg CAR and MA (1 g/L ampicillin, 500 mg/L vancomycin, 1 g/L neomycin sulfate, and 1 g/L metronidazole) (all from Aladdin) via oral gavage at 10 ml/kg each day for six weeks to induce intestinal flora disruption [20]. Rat chow was obtained from Beijing Keaoxieli Feed.
After six weeks of drug administration, all rats were subjected to a 12-hour fasting period (from 8:00 a.m. to 8:00 p.m.), during which they had access to water. Subsequently, a 0.1 ml blood sample was collected from the tail vein, and FBG was measured using an automatic blood glucose monitor (Johnson & Johnson). Following the fasting period, an oral glucose tolerance test (OGTT) and an insulin tolerance test (ITT) were conducted. For the OGTT, a 0.1 ml blood sample was collected from the tail vein after injecting rats with 2 g/kg glucose solution, and blood glucose levels were measured at 0, 30, 60, and 120 min post-injection. In the ITT, a 0.1 ml blood sample was collected from the tail vein after intraperitoneal injection with 0.75 IU/kg insulin (Novo Nordisk A/S MedChem Research), and blood glucose levels were measured at the same time intervals. Serum levels of TC (A111-1-1), low-density lipoprotein cholesterol (LDL-C, A113-1-1), high-density lipoprotein cholesterol (HDL-C, A112-1-1), and triglyceride (TG, A110-1-1) were determined using kits from Nanjing JianCheng Bioengineering Institute.
After the final blood collection, the rats were weighed. Subsequently, the rats were euthanized with an injection of 120 mg/kg pentobarbital sodium. The liver and kidneys were isolated, and their weights were measured. The hepatic or renal index was calculated as the ratio of liver weight (in milligrams) or kidney weight (in milligrams) to body weight (in grams), respectively.
Serum levels of alanine aminotransferase (ALT), aspartate aminotransferase (AST), blood urea nitrogen (BUN), creatinine (Cre), and uric acid (UA) were determined using enzyme-linked immunosorbent assay (ELISA) kits. Specifically, ALT (C009-2-1), AST (C010-1-1), BUN (C013-2-1), Cre (C011-2-1), and UA (C012-2-1) ELISA kits were obtained from Nanjing JianCheng Bioengineering Institute.
Liver and kidney tissues were paraffin-embedded and sliced into 4 µm sections. Hematoxylin and eosin (HE) staining was performed on these sections. The resulting morphological changes were visualized using a fluorescence microscope (IX-51; Olympus).
After six weeks of treatment, fresh feces samples were collected from the rats. Gas chromatography-flame ionization detector (GC-FID) analysis was used to determine the levels of SCFAs, including acetic acid, propionic acid, butyric acid, isobutyric acid, valeric acid, and isovaleric acid, in the feces [21]. Firstly, 0.1 g of feces sample was dissolved in 0.9 ml of water, mixed, and centrifuged at 13,200 rpm and 4°C for 15 min. Then, 2 µl of the supernatant was injected into the inlet for GC-FID analysis. A Perkin Elmer Clarus 80 gas chromatograph (Perkin Elmer, Inc.) was used for the analysis. The nitrogen flow rate was maintained at 2.0 ml/min. The flame ionization detector and injector were kept at 250°C. Nitrogen, hydrogen, and air flow rates were set at 19, 30, and 300 ml/min, respectively. The initial column temperature was held at 100°C for 30 sec and then increased at a rate of 4°C/min to reach 150°C.
The rat intestinal epithelial cell line IEC-6, obtained from ATCC, was cultured in Dulbecco's Modified Eagle Medium supplemented with 100 U/ml penicillin and streptomycin, 10 U/ml insulin, and 10% fetal bovine serum, under normal glucose concentrations. The cells were maintained at 37°C with 5% CO2.
IEC-6 cells were grouped as follows: the control group (5.5 mM glucose), the high glucose (HG) group (30 mM glucose + 1 nM free fatty acid [0.33 mM palmitic acid, 0.67 mM oleic acid] [22]), the HG + CAR group (cells were treated with 20, 50, 100, 200, 500 µM/ml CAR for 24 h in HG environment [16]), the HG + CAR + sodium β-hydroxybutyrate (SHB) group (cells were treated with 200 µM/ml CAR and 5 mM SHB simultaneously for 24 h in HG environment [23]), the HG + CAR + GLPG0974 (GLPG) group (cells were treated with 200 µM/ml CAR and 0.1 µM GLPG simultaneously for 24 h in HG environment [23]), the HG + SHB + GLPG group (cells were treated with 200 µM/ml CAR, 5 mM SHB and 0.1 µM GPLG simultaneously for 24 h in HG environment [23]). Among them, SHB, a GPR41 antagonist, was obtained from Sigma-Aldrich; GLPG, a GPR43 antagonist, was purchased from Tocris Bioscience [23].
Cell viability was assessed using the MTT kit (M1020; Solarbio). The optical density value was measured at 450 nm using a microplate reader (Bio-Rad 680; Bio-Rad). The specific protocol was followed as per the kit instructions.
Annexin V-FITC and propidium iodide (Beyotime) were performed on cells at room temperature for 30 min, followed by two washes with PBS. The stained cell population was analyzed using CellQuest (BD Biosciences) to collect fluorescence data.
Total RNA from rat colonic tissues and IEC-6 cells was extracted using the TRIzol method (Invitrogen). The PrimeScript RT-PCR kit (Takara) was utilized to reverse-transcribe total RNA into cDNA. The mRNA expression levels were determined on an ABI Prism 7500 Detection System (ABI) using the SYBR Premix Ex Taq kit (Takara). β-actin was selected as the internal control for assessing GPR41 and GPR43 mRNA expression. The gene primers used are listed in Table 1. The relative gene expression was calculated using the 2-ΔΔct method.
Rat colonic tissues and IEC-6 cells were homogenized using a commercial Pro-Prep Protein Extraction Solution (Beyotime). Protein concentrations were determined using the BCA kit (Beyotime) following the provided instructions. Subsequently, 30 µg of protein sample was separated by 12% SDS-PAGE and transferred onto PVDF membranes (Millipore). After blocking with 5% BSA, the membranes were incubated overnight with primary antibodies, including anti-GPR41 (1:500, PA5-75521, Thermo Fisher Scientific), anti-GPR43 (1:200, PA5-111780, Thermo Fisher Scientific), anti-cleaved-caspase-3 (1:1,000, GTX86952, GeneTex), anti-β-actin (1:1,000, ab8227, Abcam) and anti-GAPDH (1:2,000, ab181602, Abcam). Following incubation with suitable secondary antibodies, protein bands were visualized using ECL detection reagents (Thermo Fisher Scientific). The results were presented as the ratio of target protein intensity to the intensity of GAPDH and β-actin loading reference band in the same lane.
GraphPad Prism 8.01 (GraphPad Software) was employed for data analysis and graph plotting. Measurement data were expressed as mean ± standard deviation. Comparison between two sets of data was performed using the independent sample t-test, while comparison among multiple sets of data was conducted using one-way analysis of variance, followed by Tukey’s test for post-hoc analysis. Bilateral tests were employed to determine p-values, with p-values < 0.05 indicating statistical significance.
T2DM rats were induced by an 8-week high-fat diet and low-dose STZ injection. In comparison to the normal group, the T2DM group exhibited elevated FBG. However, treatment with CAR (50 mg/kg, once daily for 6 weeks) and/or MA led to decreased FBG in T2DM rats. Notably, the CAR + MA group displayed significantly increased FBG compared to the CAR group (Fig. 1A, all p < 0.05). As anticipated, OGTT and ITT results indicated higher blood glucose levels in T2DM rats compared to the normal group. Treatment with CAR reduced blood glucose levels in T2DM rats. Interestingly, blood glucose levels in the CAR + MA group were markedly elevated compared to the CAR group (Fig. 1B, C, all p < 0.05). Dyslipidemia was observed in the T2DM group, with elevated levels of TC, TG, and LDL-C levels, and decreased HDL-C levels. CAR treatment partially ameliorated these lipid imbalances in T2DM rats. However, CAR + MA treatment partially reversed the lipid-improving effects of CAR in T2DM rats (Fig. 1D, all p < 0.05). These findings demonstrated that CAR effectively lowered blood lipid and glucose levels in T2DM rats, and this effect was partially counteracted by CAR + MA treatment.
As illustrated in Fig. 2A, the liver index in the T2DM group was significantly higher than that in the normal group (p < 0.01). However, CAR treatment reduced the liver index in T2DM rats (p < 0.05). Interestingly, the liver index in the CAR + MA group was increased compared to the CAR group (p < 0.05). No significant differences were observed in kidney index among the groups (p > 0.05). Histopathological examination using HE staining revealed normal structures in liver and kidney tissues in the normal group. In contrast, T2DM rats exhibited hepatocyte swelling, glomerular proliferation, vitreous degeneration of glomerular vascular wall, and increased mesangial matrix. CAR treatment partially ameliorated liver and kidney damage in T2DM rats. Strikingly, CAR + MA treatment exacerbated liver and kidney damage (Fig. 2B). ELISA results showed elevated levels of serum ALT, AST, BUN, Cre, and UA in T2DM rats. However, CAR treatment significantly reduced these levels. Notably, serum ALT, AST, BUN, Cre, and UA levels were increased in the CAR + MA group compared to the CAR group (Fig. 2C, all p < 0.05). These findings indicated that CAR could alleviate liver and kidney tissue damage and pathological changes in T2DM rats, and this ameliorative effect was partially reversed by CAR + MA treatment.
GC-FID analysis revealed decreased levels of six SCFAs in the feces of T2DM rats compared to those in rats of the normal group. However, treatment with CAR led to increased levels of these SCFAs. Interestingly, the CAR + MA group exhibited reduced contents of these six SCFAs in rat feces compared to the CAR group (Fig. 3, all p < 0.05). Overall, these findings indicated that CAR could elevate the levels of SCFAs in the feces of T2DM rats.
RT-qPCR analysis demonstrated that the mRNA expression of the SCFA receptors GPR41 and GPR43 mRNA was downregulated in colonic tissues of T2DM rats. However, CAR treatment resulted in an upregulation of GPR41 and GPR43 mRNA expression. In contrast, the CAR + MA group displayed decreased GPR41 and GPR43 mRNA levels compared to the CAR group (Fig. 4A, all p < 0.01). Western blot analysis showed similar tendencies in GPR41 and GPR43 protein levels among the normal, T2DM, CAR, and CAR + MA groups (Fig. 4B, all p < 0.05). These findings indicated that CAR could activate the expression of GPR41/43 in colonic tissues of T2DM rats.
Subsequently, IEC-6 cells were exposed to a HG environment and treated with various concentrations of CAR (20, 50, 100, 200, and 500 µM/ml). MTT results indicated that cell viability was reduced in the HG group compared to the control group (Fig. 5A, p < 0.001). Low to medium doses of CAR (20–200 µM/ml) increased the viability of HG-induced IEC-6 cells, with a dose-dependent effect (Fig. 5A, all p < 0.05). Although 500 µM/ml CAR caused a slight decrease in cell viability compared to 200 µM/ml CAR, the difference was not significant (Fig. 5A, p > 0.05). Therefore, 200 µM/ml CAR was selected for subsequent experiments involving HG-induced IEC-6 cells. To explore the relationship between CAR and GPR41/43 expression, HG-induced IEC-6 cells were treated with CAR alone or in combination with SHB and/or GPLG, antagonists of GPR41 and GPR43, respectively. Flow cytometry, RT-qPCR and Western blot results showed that apoptotic rate and cleaved-caspase-3 protein levels were up-regulated, while GPR41 and GPR43 mRNA and protein levels were downregulated in the HG group compared to the control group. In contrast, the HG + CAR group exhibited decreased apoptotic rates and cleaved-caspase-3 protein levels, along with increased GPR41 and GPR43 mRNA and protein levels. Inhibition of GPR41 or GPR43 expression partially reversed these changes. Additionally, the HG + CAR + SHB + GPLG group displayed elevated apoptotic rates and cleaved-caspase-3 protein levels, as well as significantly reduced GPR41 and GPR43 mRNA and protein levels group compared to the HG + CAR + SHB group and the HG + CAR + GPLG group (Fig. 5B–D, all p < 0.05). These findings collectively indicated that CAR treatment suppressed HG-induced apoptosis of IEC-6 cells and concurrently activated GPR41/43 expression.
T2DM presents a significant health challenge due to its associated complications and increased morbidity and mortality rates, including microvascular and macrovascular events [24]. Natural compounds are being explored for their potential to impact intestinal flora and gut microbiota-mediated SCFA metabolism, offering advantages of low doses and mild side effects [25]. CAR has shown promising effects in the context of diabetes pathology [26]. This study unveils, for the first time, that CAR can alleviate blood lipid and glucose levels in T2DM rats by regulating SCFAs and mediating the GPR41/43 pathway.
Maintaining controlled blood glucose and lipid levels is pivotal for preventing or delaying complications in diabetes management [27]. Elevated FBG is a hallmark challenge in T2DM patients and serves as an indicator of glycemic control [28]. Our findings demonstrate elevated FBG levels in T2DM rats, which were reduced following CAR treatment. The overall blood glucose trends mirrored the changes in FBG. Dyslipidemia, characterized by increased levels of LDL-C and TG, along with decreased HDL-C levels, is a common comorbidity in T2DM patients, and elevated TC is linked to T2DM susceptibility [29]. In our T2DM rat model, TC, TG, and LDL-C levels were elevated, while HDL-C levels were reduced. After administering CAR, there was a reversal in the trends of TC, TG, LDL-C, and HDL-C in T2DM rats. Previous studies have also indicated that CAR can significantly reduce blood glucose levels in T2DM mice and alleviate dyslipidemia in db/db mice [30,31]. In light of these collective findings, it can be concluded that CAR exerts a positive effect on blood lipid and glucose regulation in T2DM rats.
T2DM can have adverse effects on liver and kidney functions, potentially leading tissue damage and pathological changes in these organs [32,33]. Our results indicate that CAR treatment reduced the liver index and ameliorated liver and kidney damage in T2DM rats. The production of UA is closely association with liver and kidney functions [34]. Liver damage can lead to the release of significant amounts of AST and ALT into the bloodstream [35]. Elevated Cre levels signify glomerular filtration dysfunction [36]. CAR-treated T2DM rats exhibited decreased levels of ALT, AST, BUN, Cre, and UA in their sera. Previous studies have also indicated CAR's potential to alleviate histopathological liver damage in T2DM db/db mice [31]. Furthermore, CAR has demonstrated its ability to mitigate kidney damage induced by acetaminophen in male rats [37]. Collectively, these findings highlight CAR's potential to alleviate liver and kidney tissue damage in the context of T2DM.
Decreased levels of SCFA due to a reduction in SCFA-producing bacteria abundance have been implicated in promoting the development of T2DM [13]. Our study revealed that CAR treatment led to an increase in the contents of six SCFAs in the feces of T2DM rats. Modifications in antibiotic usage can influence the gut microbiome [19], and in our study, the administration of MA reversed the effect of CAR on SCFAs in the feces of T2DM rats. Phytogenic supplements containing menthol, CAR, and carvone have been shown to alleviate gut microbiota imbalances [18]. Based on these observations, it can be inferred that CAR contributes to higher SCFA levels in T2DM rats. Notably, SCFAs such as propionate, acetate, and butyrate differentially activate GPR41 and GPR43, which in turn can inhibit the synthesis of hepatic cholesterol and fatty acids, and play a role in modulating obesity in mice [38]. Furthermore, decreased levels of GPR41/43 receptors have been observed in placenta tissues from gestational diabetes mellitus cases [39]. In our study, CAR administration led to an upregulation of GPR41 and GPR43 levels in the colonic tissues of T2DM rats, an effect that was nullified by MA treatment. Collectively, these findings suggest that CAR can enhance the expression of GPR41 and GPR43 receptors in the context of T2DM.
In summary, this study illuminates the potential of CAR in ameliorating blood lipid and glucose levels in T2DM rats through the modulation of SCFAs and the GPR41/43 pathway. However, several limitations warrant consideration. Firstly, our analysis of SCFA content was confined to fecal samples, lacking a more comprehensive exploration of intestinal flora species and abundance using 16S rDNA high-throughput sequencing technology. This approach could provide deeper insights into the specific mechanisms by which CAR influences the intestinal flora of T2DM rats, including the receptors, DNA, and proteins that CAR interacts with to regulate SCFA levels, ultimately leading to improved lipid and blood glucose profiles. Additionally, our study employed a relatively short intervention period of six weeks, and the long-term therapeutic efficacy of CAR remains unverified. Furthermore, only MA was used to disrupt the intestinal flora of mice, potentially warranting the investigation of other MA regimens in rats. As such, future studies should consider adopting 16S rDNA high-throughput sequencing technology to comprehensively analyze intestinal flora species and abundance, further refining the obtained results. Prolonged intervention periods should be explored to assess CAR's long-term impact on T2DM, and additional investigation of MA dosing and timing in rats would enhance the understanding of the role of gut microbiota in CAR's effects.
REFERENCES
1. Artasensi A, Pedretti A, Vistoli G, Fumagalli L. 2020; Type 2 diabetes mellitus: a review of multi-target drugs. Molecules. 25:1987. DOI: 10.3390/molecules25081987. PMID: 32340373. PMCID: PMC7221535. PMID: bbe44d47ab4e4b0db145ac2f3112f432.
2. Wu Y, Ding Y, Tanaka Y, Zhang W. 2014; Risk factors contributing to type 2 diabetes and recent advances in the treatment and prevention. Int J Med Sci. 11:1185–1200. DOI: 10.7150/ijms.10001. PMID: 25249787. PMCID: PMC4166864.
3. Sun H, Saeedi P, Karuranga S, Pinkepank M, Ogurtsova K, Duncan BB, Stein C, Basit A, Chan JCN, Mbanya JC, Pavkov ME, Ramachandaran A, Wild SH, James S, Herman WH, Zhang P, Bommer C, Kuo S, Boyko EJ, Magliano DJ. 2022; IDF Diabetes Atlas: global, regional and country-level diabetes prevalence estimates for 2021 and projections for 2045. Diabetes Res Clin Pract. 183:109119. DOI: 10.1016/j.diabres.2021.109119. PMID: 34879977.
4. Landgraf R, Aberle J, Birkenfeld AL, Gallwitz B, Kellerer M, Klein H, Müller-Wieland D, Nauck MA, Reuter HM, Siegel E. 2019; Therapy of type 2 diabetes. Exp Clin Endocrinol Diabetes. 127:S73–S92. DOI: 10.1055/a-1018-9106. PMID: 31860927.
5. Brunkwall L, Orho-Melander M. 2017; The gut microbiome as a target for prevention and treatment of hyperglycaemia in type 2 diabetes: from current human evidence to future possibilities. Diabetologia. 60:943–951. DOI: 10.1007/s00125-017-4278-3. PMID: 28434033. PMCID: PMC5423958.
6. de Almeida Duarte JB, de Aguilar-Nascimento JE, Nascimento M, Nochi RJ Jr. 2004; Bacterial translocation in experimental uremia. Urol Res. 32:266–270. DOI: 10.1007/s00240-003-0381-7. PMID: 15497213.
7. Gonçalves S, Pecoits-Filho R, Perreto S, Barberato SH, Stinghen AE, Lima EG, Fuerbringer R, Sauthier SM, Riella MC. 2006; Associations between renal function, volume status and endotoxaemia in chronic kidney disease patients. Nephrol Dial Transplant. 21:2788–2794. DOI: 10.1093/ndt/gfl273. PMID: 16861246.
8. Magnusson M, Magnusson KE, Sundqvist T, Denneberg T. 1991; Impaired intestinal barrier function measured by differently sized polyethylene glycols in patients with chronic renal failure. Gut. 32:754–759. DOI: 10.1136/gut.32.7.754. PMID: 1855681. PMCID: PMC1378990.
9. Dalile B, Van Oudenhove L, Vervliet B, Verbeke K. 2019; The role of short-chain fatty acids in microbiota-gut-brain communication. Nat Rev Gastroenterol Hepatol. 16:461–478. DOI: 10.1038/s41575-019-0157-3. PMID: 31123355.
10. Blaak EE, Canfora EE, Theis S, Frost G, Groen AK, Mithieux G, Nauta A, Scott K, Stahl B, van Harsselaar J, van Tol R, Vaughan EE, Verbeke K. 2020; Short chain fatty acids in human gut and metabolic health. Benef Microbes. 11:411–455. DOI: 10.3920/BM2020.0057. PMID: 32865024.
11. Gowd V, Xie L, Zheng X, Chen W. 2019; Dietary fibers as emerging nutritional factors against diabetes: focus on the involvement of gut microbiota. Crit Rev Biotechnol. 39:524–540. DOI: 10.1080/07388551.2019.1576025. PMID: 30810398.
12. Ratajczak W, Rył A, Mizerski A, Walczakiewicz K, Sipak O, Laszczyńska M. 2019; Immunomodulatory potential of gut microbiome-derived short-chain fatty acids (SCFAs). Acta Biochim Pol. 66:1–12. DOI: 10.18388/abp.2018_2648. PMID: 30831575.
13. Zhao L, Zhang F, Ding X, Wu G, Lam YY, Wang X, Fu H, Xue X, Lu C, Ma J, Yu L, Xu C, Ren Z, Xu Y, Xu S, Shen H, Zhu X, Shi Y, Shen Q, Dong W, et al. 2018; Gut bacteria selectively promoted by dietary fibers alleviate type 2 diabetes. Science. 359:1151–1156. DOI: 10.1126/science.aao5774. PMID: 29590046.
14. Parada Venegas D, De la Fuente MK, Landskron G, González MJ, Quera R, Dijkstra G, Harmsen HJM, Faber KN, Hermoso MA. 2019; Short Chain Fatty Acids (SCFAs)-mediated gut epithelial and immune regulation and its relevance for inflammatory bowel diseases. Front Immunol. 10:277. Erratum in: Front Immunol. 2019;10: 1486. DOI: 10.3389/fimmu.2019.01486. PMID: 31316522. PMCID: PMC6611342. PMID: a9169df15629429c9d20f7960e3fbb21.
15. Sharifi-Rad M, Varoni EM, Iriti M, Martorell M, Setzer WN, Del Mar Contreras M, Salehi B, Soltani-Nejad A, Rajabi S, Tajbakhsh M, Sharifi-Rad J. 2018; Carvacrol and human health: a comprehensive review. Phytother Res. 32:1675–1687. DOI: 10.1002/ptr.6103. PMID: 29744941.
16. Zhao W, Deng C, Han Q, Xu H, Chen Y. 2020; Carvacrol may alleviate vascular inflammation in diabetic db/db mice. Int J Mol Med. 46:977–988. Erratum in: Int J Mol Med. 2023;52:90. DOI: 10.3892/ijmm.2020.4654. PMID: 32583003. PMCID: PMC7388828.
17. Bayramoglu G, Senturk H, Bayramoglu A, Uyanoglu M, Colak S, Ozmen A, Kolankaya D. 2014; Carvacrol partially reverses symptoms of diabetes in STZ-induced diabetic rats. Cytotechnology. 66:251–257. DOI: 10.1007/s10616-013-9563-5. PMID: 23579248. PMCID: PMC3918264.
18. Bajagai YS, Petranyi F, J Yu S, Lobo E, Batacan R Jr, Kayal A, Horyanto D, Ren X, M Whitton M, Stanley D. 2022; Phytogenic supplement containing menthol, carvacrol and carvone ameliorates gut microbiota and production performance of commercial layers. Sci Rep. 12:11033. DOI: 10.1038/s41598-022-14925-0. PMID: 35773309. PMCID: PMC9246849. PMID: 036b1d04b47b4e7396149427618d0108.
19. Zeng Z, Guo X, Zhang J, Yuan Q, Chen S. 2021; Lactobacillus paracasei modulates the gut microbiota and improves inflammation in type 2 diabetic rats. Food Funct. 12:6809–6820. DOI: 10.1039/D1FO00515D. PMID: 34113945.
20. Rakoff-Nahoum S, Paglino J, Eslami-Varzaneh F, Edberg S, Medzhitov R. 2004; Recognition of commensal microflora by toll-like receptors is required for intestinal homeostasis. Cell. 118:229–241. DOI: 10.1016/j.cell.2004.07.002. PMID: 15260992.
21. Xiao S, Liu C, Chen M, Zou J, Zhang Z, Cui X, Jiang S, Shang E, Qian D, Duan J. 2020; Scutellariae radix and coptidis rhizoma ameliorate glycolipid metabolism of type 2 diabetic rats by modulating gut microbiota and its metabolites. Appl Microbiol Biotechnol. 104:303–317. DOI: 10.1007/s00253-019-10174-w. PMID: 31758238.
22. Li R, Ye Z, She D, Fang P, Zong G, Hu K, Kong D, Xu W, Li L, Zhou Y, Zhang K, Xue Y. 2022; Semaglutide may alleviate hepatic steatosis in T2DM combined with NFALD mice via miR-5120/ABHD6. Drug Des Devel Ther. 16:3557–3572. DOI: 10.2147/DDDT.S384884. PMID: 36238196. PMCID: PMC9553160.
23. Li M, van Esch BCAM, Henricks PAJ, Folkerts G, Garssen J. 2018; The anti-inflammatory effects of short chain fatty acids on lipopolysaccharide- or tumor necrosis factor α-stimulated endothelial cells via activation of GPR41/43 and inhibition of HDACs. Front Pharmacol. 9:533. DOI: 10.3389/fphar.2018.00533. PMID: 29875665. PMCID: PMC5974203. PMID: a1f4016aed6c4578972d7f5803470619.
24. Ortiz-Martínez M, González-González M, Martagón AJ, Hlavinka V, Willson RC, Rito-Palomares M. 2022; Recent developments in biomarkers for diagnosis and screening of type 2 diabetes mellitus. Curr Diab Rep. 22:95–115. DOI: 10.1007/s11892-022-01453-4. PMID: 35267140. PMCID: PMC8907395.
25. He L, Yang FQ, Tang P, Gao TH, Yang CX, Tan L, Yue P, Hua YN, Liu SJ, Guo JL. 2022; Regulation of the intestinal flora: a potential mechanism of natural medicines in the treatment of type 2 diabetes mellitus. . Biomed Pharmacother. 151:113091. DOI: 10.1016/j.biopha.2022.113091. PMID: 35576662.
26. Cicalău GIP, Babes PA, Calniceanu H, Popa A, Ciavoi G, Iova GM, Ganea M, Scrobotă I. 2021; Anti-inflammatory and antioxidant properties of carvacrol and magnolol, in periodontal disease and diabetes mellitus. Molecules. 26:6899. DOI: 10.3390/molecules26226899. PMID: 34833990. PMCID: PMC8623889. PMID: 296660bef2f74cfca7bf81f983503945.
27. Pradeepa R, Mohan V. 2021; Epidemiology of type 2 diabetes in India. Indian J Ophthalmol. 69:2932–2938. DOI: 10.4103/ijo.IJO_1627_21. PMID: 34708726. PMCID: PMC8725109.
28. Wang LL, Wang Q, Hong Y, Ojo O, Jiang Q, Hou YY, Huang YH, Wang XH. 2018; The effect of low-carbohydrate diet on glycemic control in patients with type 2 diabetes mellitus. Nutrients. 10:661. DOI: 10.3390/nu10060661. PMID: 29882884. PMCID: PMC6024764. PMID: 78aed7886f48488db79273c57b96e277.
29. Soremekun O, Karhunen V, He Y, Rajasundaram S, Liu B, Gkatzionis A, Soremekun C, Udosen B, Musa H, Silva S, Kintu C, Mayanja R, Nakabuye M, Machipisa T, Mason A, Vujkovic M, Zuber V, Soliman M, Mugisha J, Nash O, et al. 2022; Lipid traits and type 2 diabetes risk in African ancestry individuals: a Mendelian Randomization study. EBioMedicine. 78:103953. DOI: 10.1016/j.ebiom.2022.103953. PMID: 35325778. PMCID: PMC8941323.
30. Hou N, Mai Y, Qiu X, Yuan W, Li Y, Luo C, Liu Y, Zhang G, Zhao G, Luo JD. 2019; Carvacrol attenuates diabetic cardiomyopathy by modulating the PI3K/AKT/GLUT4 pathway in diabetic mice. Front Pharmacol. 10:998. DOI: 10.3389/fphar.2019.00998. PMID: 31572181. PMCID: PMC6751321. PMID: 3a2d1f3f6cfe422abb1231e3479394e9.
31. Zhao W, Chen L, Zhou H, Deng C, Han Q, Chen Y, Wu Q, Li S. 2021; Protective effect of carvacrol on liver injury in type 2 diabetic db/db mice. Mol Med Rep. 24:741. DOI: 10.3892/mmr.2021.12381. PMID: 34435648. PMCID: PMC8430346.
32. Hadi A, Arab A, Hajianfar H, Talaei B, Miraghajani M, Babajafari S, Marx W, Tavakoly R. 2020; The effect of fenugreek seed supplementation on serum irisin levels, blood pressure, and liver and kidney function in patients with type 2 diabetes mellitus: a parallel randomized clinical trial. Complement Ther Med. 49:102315. DOI: 10.1016/j.ctim.2020.102315. PMID: 32147060.
33. Zhou N, Zhao Y, Zhang L, Ning Y. 2022; Protective effects of black onion polysaccharide on liver and kidney injury in T2DM rats through the synergistic impact of hypolipidemic and antioxidant abilities. Int J Biol Macromol. 223:378–390. DOI: 10.1016/j.ijbiomac.2022.11.055. PMID: 36368355.
34. Li L, Li Y, Luo J, Jiang Y, Zhao Z, Chen Y, Huang Q, Zhang L, Wu T, Pang J. 2021; Resveratrol, a novel inhibitor of GLUT9, ameliorates liver and kidney injuries in a D-galactose-induced ageing mouse model via the regulation of uric acid metabolism. Food Funct. 12:8274–8287. DOI: 10.1039/D1FO00538C. PMID: 34180933.
35. Sampath Kumar A, Maiya AG, Shastry BA, Vaishali K, Ravishankar N, Hazari A, Gundmi S, Jadhav R. 2019; Exercise and insulin resistance in type 2 diabetes mellitus: a systematic review and meta-analysis. Ann Phys Rehabil Med. 62:98–103. DOI: 10.1016/j.rehab.2018.11.001. PMID: 30553010.
36. Medler S, Harrington F. 2013; Measuring dynamic kidney function in an undergraduate physiology laboratory. Adv Physiol Educ. 37:384–391. DOI: 10.1152/advan.00057.2013. PMID: 24292917.
37. Najafizadeh A, Kaeidi A, Rahmani M, Hakimizadeh E, Hassanshahi J. 2022; The protective effect of carvacrol on acetaminophen-induced renal damage in male rats. Mol Biol Rep. 49:1763–1771. DOI: 10.1007/s11033-021-06985-8. PMID: 35020122.
38. Zaky A, Glastras SJ, Wong MYW, Pollock CA, Saad S. 2021; The role of the gut microbiome in diabetes and obesity-related kidney disease. Int J Mol Sci. 22:9641. DOI: 10.3390/ijms22179641. PMID: 34502562. PMCID: PMC8431784. PMID: 4429670807454178b0379913dc0763d2.
39. Wang S, Liu Y, Qin S, Yang H. 2022; Composition of maternal circulating short-chain fatty acids in gestational diabetes mellitus and their associations with placental metabolism. Nutrients. 14:3727. DOI: 10.3390/nu14183727. PMID: 36145103. PMCID: PMC9505713. PMID: f97ae44b2c974540a779fe52f8b24c58.
Fig. 1
CAR reduces blood lipid and glucose levels in T2DM rats.
(A) FBG levels. (B) OGTT results. (C) ITT results. (D) Levels of serum TC, TG, LDL-C, and HDL-C. N = 6. The data were described as mean ± standard deviation. Multiple groups of data were compared using one-way analysis of variance, followed by Tukey’s test. CAR, carvacrol; T2DM, type 2 diabetes mellitus; MA, mixed antibiotics; FBG, fasting blood glucose; OGTT, oral glucose tolerance test; ITT, insulin tolerance test; TC, total cholesterol; TG, triglyceride; LDL-C, low-density lipoprotein cholesterol; HDL-C, high-density lipoprotein cholesterol. *p < 0.05, **p < 0.01.
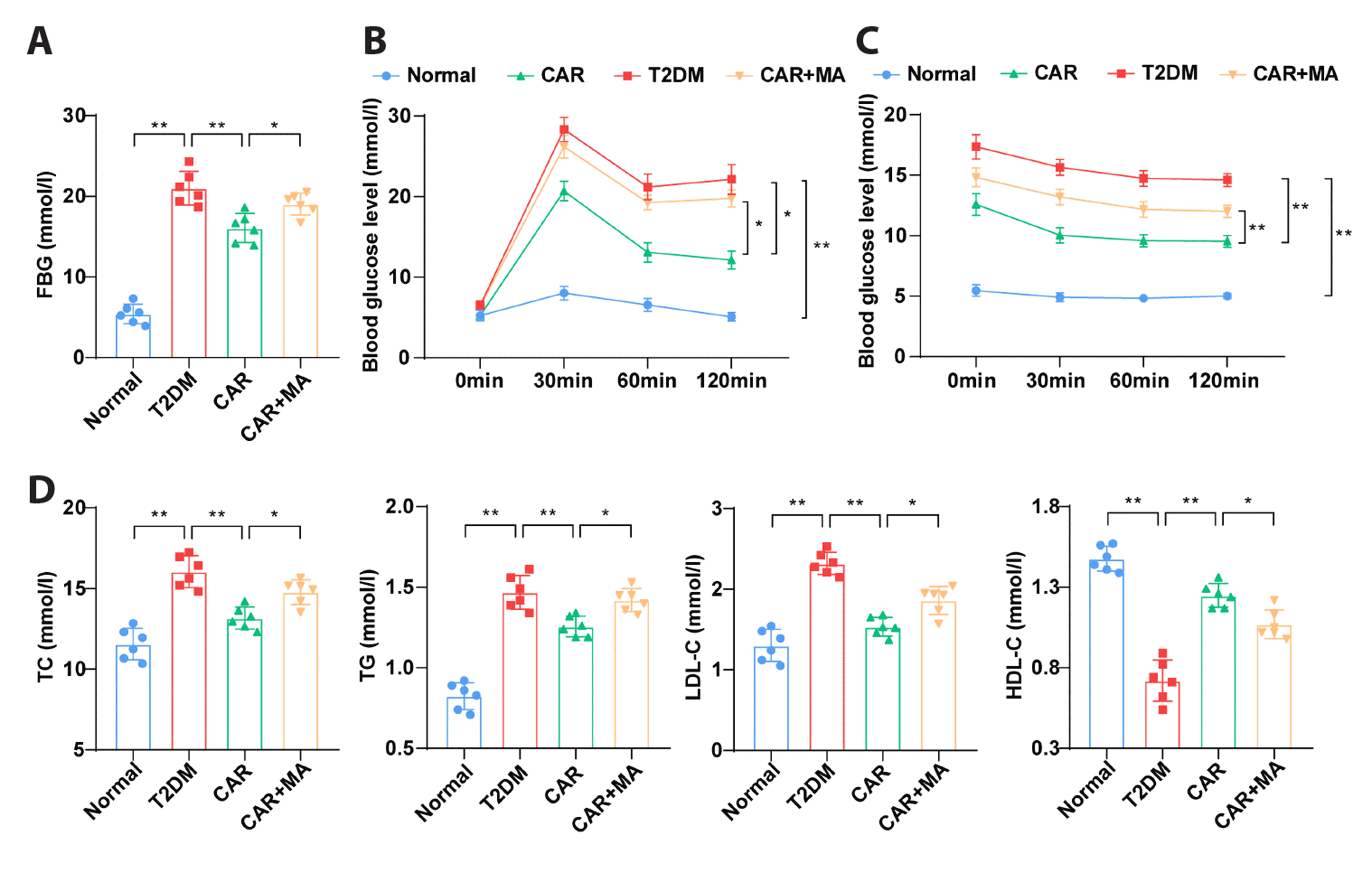
Fig. 2
CAR alleviates liver and kidney tissue damage and pathological changes in T2DM rats.
(A) Liver and kidney indexes. (B) The pathological changes in rat liver and kidney tissues observed by HE staining. (C) Levels of ALT, AST, BUN, Cre, and UA measured by ELISA. N = 6. The data were described as mean ± standard deviation. Multiple groups of data were compared using one-way analysis of variance, followed by Tukey’s test. CAR, carvacrol; T2DM, type 2 diabetes mellitus; MA, mixed antibiotics; HE, hematoxylin and eosin; ALT, alanine aminotransferase; AST, aspartate aminotransferase; BUN, blood urea nitrogen; Cre, creatinine; UA, uric acid. nsp > 0.05, *p < 0.05, **p < 0.01.
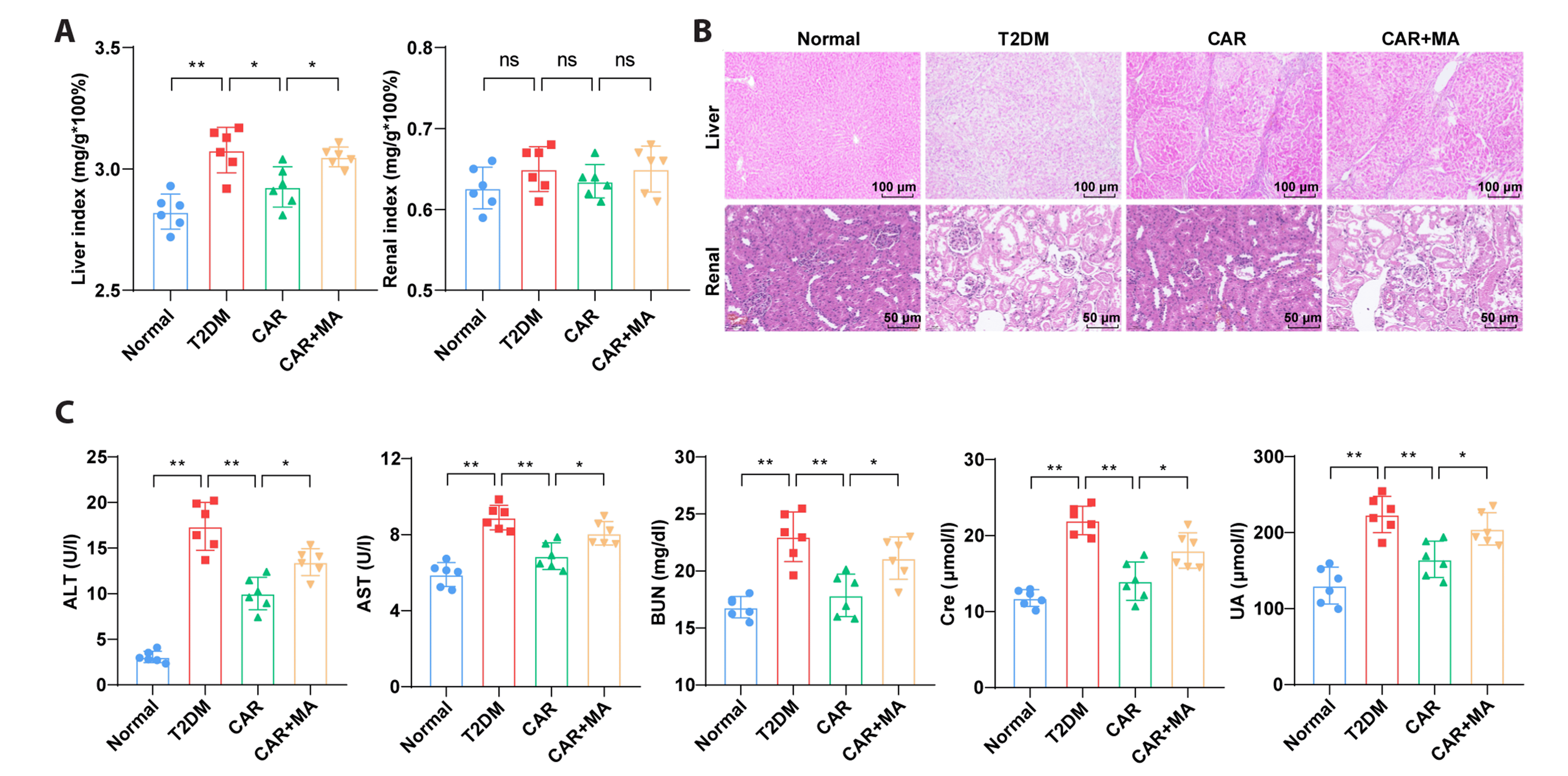
Fig. 3
CAR increases levels of SCFAs in feces of T2DM rats.
(A) Acetic acid. (B) Propionic acid. (C) Butyric acid. (D) Isobutyric acid. (E) Valeric acid. (F) Isovaleric acid. N = 6. The data were described as mean ± standard deviation. Multiple groups of data were compared using one-way analysis of variance, followed by Tukey’s test. CAR, carvacrol; SCFAs, short-chain fatty acids; T2DM, type 2 diabetes mellitus; MA, mixed antibiotics. *p < 0.05, **p < 0.01.
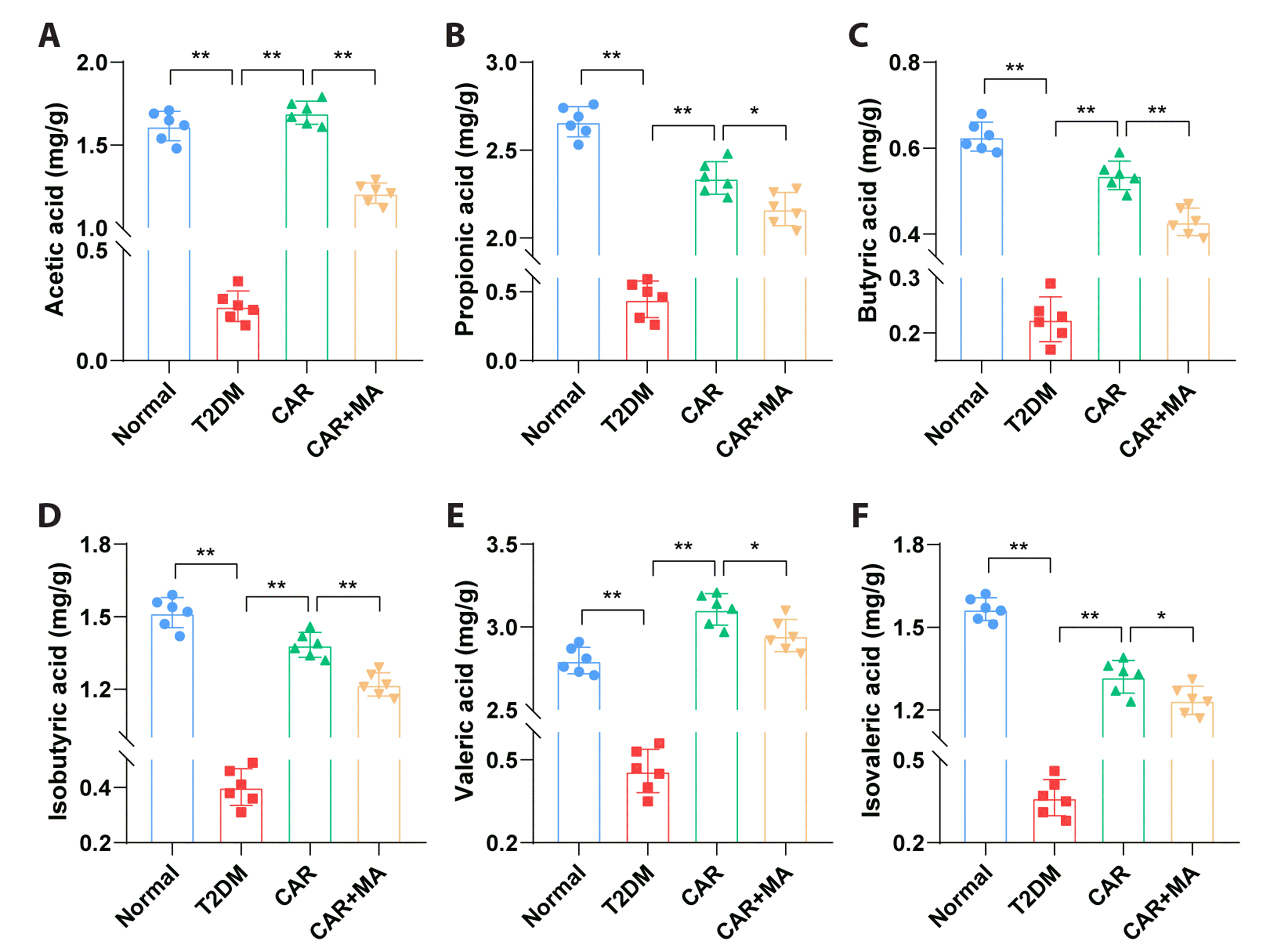
Fig. 4
CAR stimulates the expression of GPR41/43 in colonic tissues of T2DM rats.
(A) The mRNA levels of GPR41 and GPR43 in colonic tissues measured by RT-qPCR. (B) The protein levels of GPR41 and GPR43 in colonic tissues measured by Western blot. N = 6. The data were described as mean ± standard deviation. Multiple groups of data were compared using one-way analysis of variance, followed by Tukey’s test. CAR, carvacrol; T2DM, type 2 diabetes mellitus; MA, mixed antibiotics; RT-qPCR, reverse transcription-quantitative polymerase chain reaction. *p < 0.05, **p < 0.01.
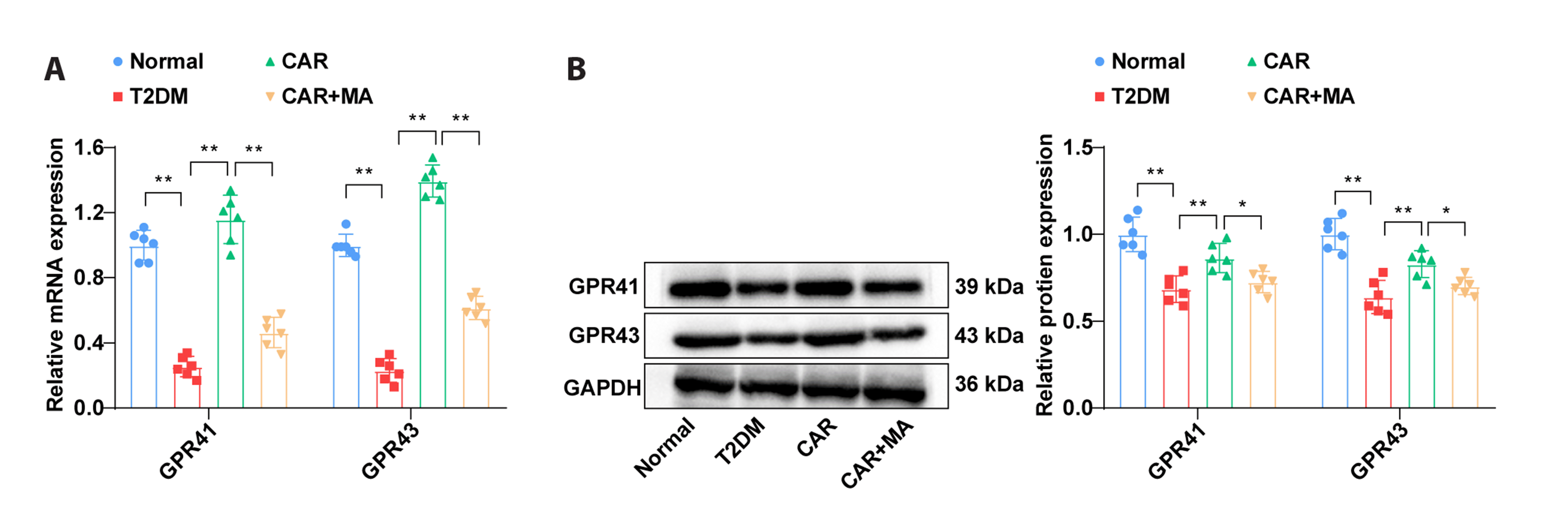
Fig. 5
CAR inhibits HG-induced apoptosis in IEC-6 cells and activates GPR41/43 expression.
(A) The optimal concentration of CAR was determined. (B) Apoptosis was assessed by flow cytometry. (C) Cleaved-caspase-3, GPR41 and GPR43 protein expression levels were assessed by Western blot. (D) GPR41 and GPR43 mRNA levels in cells were determined by RT-qPCR. Cell experiments were repeated three times and data are expressed as mean ± standard deviation. One-way analysis of variance was utilized for data comparisons among multiple groups. Tukey’s multiple comparisons test was applied for post-hoc tests. CAR, carvacrol; HG, high glucose; RT-qPCR, reverse transcription-quantitative polymerase chain reaction; Con, control; SHB, sodium β-hydroxybutyrate; GLPG, GLPG0974; nsp >0.05. *p < 0.05, **p < 0.01, ***p < 0.001.
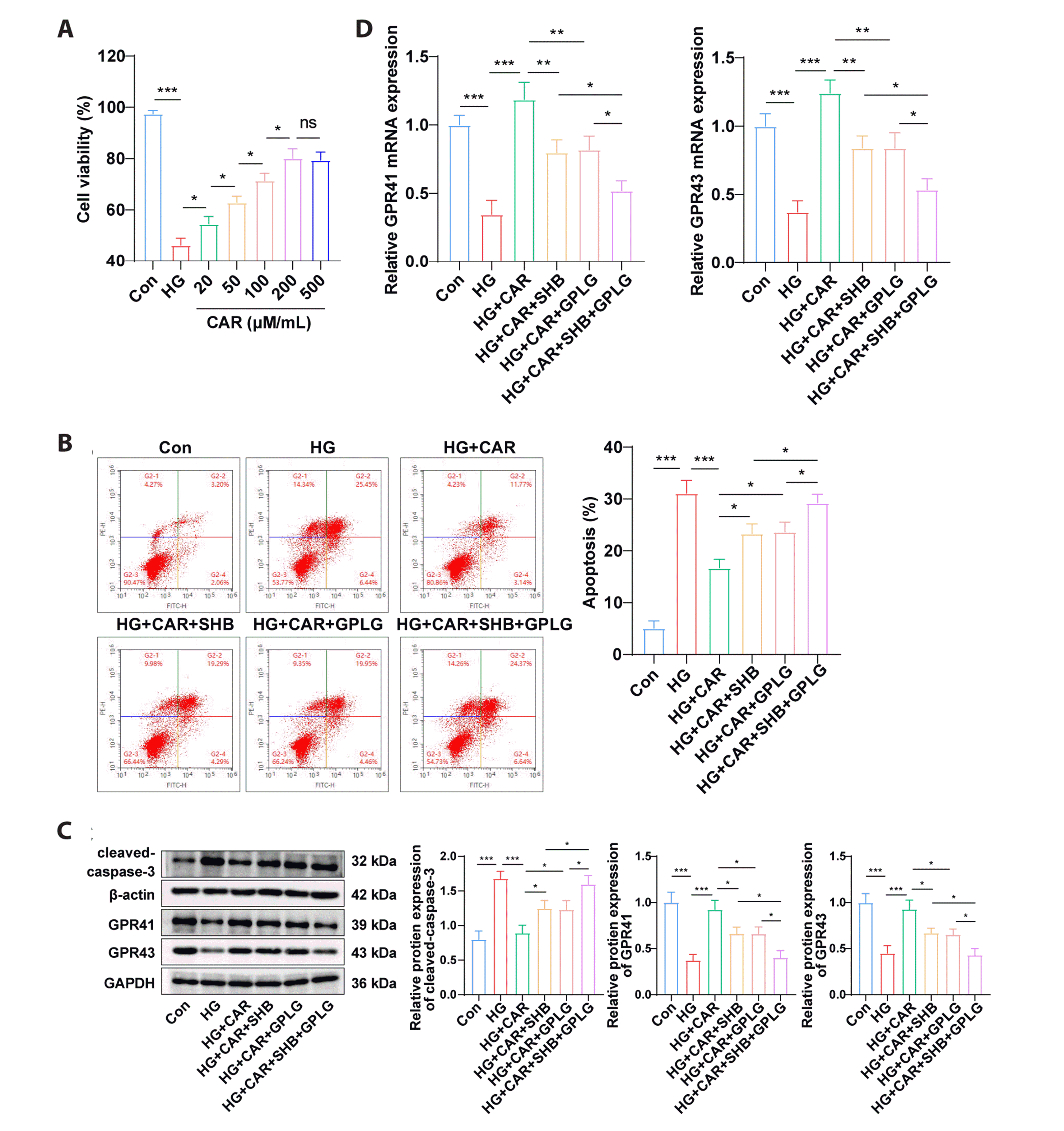