Abstract
PURPOSE
The purpose of this study was to evaluate the properties of a porous zirconia scaffold coated with bioactive materials and compare the in vitro cellular behavior of MC3T3-E1 preosteoblastic cells to titanium and zirconia disks and porous zirconia scaffolds.
MATERIALS AND METHODS
Titanium and zirconia disks were prepared. A porous zirconia scaffold was fabricated with an open cell polyurethane disk foam template. The porous zirconia scaffolds were coated with β-TCP, HA and a compound of β-TCP and HA (BCP). The characteristics of the specimens were evaluated using scanning electron microscopy (SEM), energy dispersive x-ray spectrometer (EDX), and x-ray diffractometry (XRD). The dissolution tests were analyzed by an inductively coupled plasma spectrometer (ICP). The osteogenic effect of MC3T3-E1 cells was assessed via cell counting and reverse transcriptase-polymerase chain reaction (RT-PCR).
RESULTS
The EDX profiles showed the substrate of zirconia, which was surrounded by the Ca-P layer. In the dissolution test, dissolved Ca2+ ions were observed in the following decreasing order; β-TCP > BCP > HA (P<.05). In the cellular experiments, the cell proliferation on titanium disks appeared significantly lower in comparison to the other groups after 5 days (P<.05). The zirconia scaffolds had greater values than the zirconia disks (P<.05). The mRNA level of osteocalcin was highest on the non-coated zirconia scaffolds after 7 days.
Dental implants are used more widely for the restoration of edentulous patients in recent years. Many studies have been conducted on the long-term stability of osseointegrated dental implants.1 As dental implants have gained popularity, the necessity for bone grafts has become more prevalent. In many cases, the quantity of alveolar bone is often insufficient for the insertion of dental implant, because in many cases the tooth loss due to periodontitis or trauma is accompanied with alveolar bone loss. In addition, bone loss can occur due to peri-implantitis after implantation. Therefore, bone grafting procedures are becoming increasingly common.2,3
There are four classifications of bone graft materials. These four types are known as autogenous bones, allogeneic bones, xenogeneic bones and alloplastic materials.4 Among these materials, autogenous bones are the safest from immune rejection, but require additional surgical procedures for harvesting from donors. Allogeneic and xenogeneic bones require no additional procedures. However, there is a risk of disease transmission and it is impossible to adjust rates of biodegradation. On the other hands, for alloplastic materials, it is possible to regulate the rates of biodegradation and much easier to be sculpted into desired three dimensional forms.5,6 There have been several materials used for alloplastic materials. Hydroxyapatite (HA), calcium phosphate, corals and bioglass are popular alloplastic bone graft materials.7 Tricalcium phosphate (TCP, Ca3(PO4)2), which has a Ca/P molar ratio of 1.5 has been used in clinical trials. It has the crystal forms of alpha and beta, and β state shows greater microporosity and degradation than hydroxyapatite.8 HA is the most widely used material. A molecular formula of HA is Ca10(PO4)6(OH)2, and its Ca/P ratio of 1.67 is most similar to the human bone structure. The HA is an osteoconductive replacement material studied by numerous investigations, whether in vitro or in vivo. The results from clinical trials have showed that HA is widely used clinically.9,10 Biphasic calcium phosphate (BCP) is a mixture of tricalcium phosphate and hydroxyapatite. Researches have reported that BCPs provide great biocompatibility and osteoconduction.11,12 In particular, mixtures of 40% β-tricalcium phosphate (β-TCP) and 60% hydroxyapatite produce better results than other compounds.13,14
Alloplastic materials can be made in the desired three dimensional forms, including a porous structure. The porous structure of bone graft materials is advantageous for the formation of new bone. However, mechanical strength is a limiting factor in porous structures. In order to overcome this mechanical defect, porous scaffolds have been made of hard materials such as alumina or zirconia and coated with bioactive materials.15 Hard scaffolds serve to maintain the volume of graft materials and therefore are useful in vertical bone augmentation.
Zirconium dioxide is commonly called zirconia. Zirconia adopts a tetragonal structure at high temperature and a monoclinic crystal at room temperature. The volume expansion caused by cooling from high temperature results in cracking. In order to stabilize the tetragonal phase, zirconia is blended with a metal oxide such as yttria (Y2O3). This is called tetragonal zirconia polycrystals (TZP). In particular, yttria stabilized TZP (Y-TZP) has superior biocompatibility and mechanical properties in comparison to other TZPs.16 Accordingly, it has been used as a material in artificial joints for a long time.17,18,19 Some studies on the biocompatibility of zirconia showed better results at osseointegration than titanium.20,21 Due to these properties of zirconia, it is useful as a scaffold for bone graft material.
While osseointegration and the mechanical properties of zirconia have been relatively much investigated, there are a few studies on the osteoblastic response of porous zirconia.
In this study, the porous zirconia scaffolds coated with CaP ceramics were fabricated using the powder slurry method. The purpose of this study was to assess whether the coating procedures were adequate and to evaluate the biochemical properties of porous zirconia scaffolds coated with bioactive materials such as their dissolution behaviors. And another aim of this study was to investigate the response of osteoblast-like cells cultured on the titanium and zirconia disks and porous zirconia scaffold by observing cell attachment and proliferation, and gene expression of the osteoblastic cell using Mus musculus (mouse) calvaria derived osteoblast precursor cell (MC3T3-E1).
The titanium disks were fabricated from grade IV commercially pure titanium (Dynamet, Carpenter Technology Co., Washington, PA, USA), 8 mm in diameter and 4 mm in thickness. Pre-sintered zirconia blocks (LAVA™ Zirconia Block, 3M ESPE, Neuss, Germany) were designed and sintered to be 8 mm in diameter and 4 mm in thickness.
An open cell polyurethane disk foam template (∅10 mm × 5 mm, 60 ppi, Filtrocell, Eurofoam, Troisdorf, Germany) was used to fabricate a porous scaffold. The polyurethane foam was dipped in 2% NaOH solution for 30 minutes and washed with distilled water and dried at room temperature for 24 hours. 100 g of ZrO2 powder (TZ-3Y-E, TOSOH Co., Yamaguchi, Japan) calcined at 600℃ for 2 hours and 6 g of triethyl phosphate (TEP) were mixed in ethanol for 24 hours by a ball mill. The slurry was completed by adding 6 g of polyvinyl butyral (PVB). It was stirred for 24 hours. Pretreated polyurethane foams were dipped and compressed several times in the slurry. And residual slurry was removed by using at low speed by centrifuge. Coated foams were dried at 60℃ for 10 minutes. After this procedure was repeated two times, the coated foams were dried at room temperature for 24 hours. The coated foams were sintered at a heating ratio of 1℃/min to 600℃, and then maintained at 600℃ for 2 hours in order for the polyurethane foams to be burnt out. After that, heat-treatment was performed at a heating ratio of 3℃/min to 1550℃ for solidification. β-TCP powders (β-tricalcium phosphate, Sigma-Aldrich Corp., St. Louis, MO, USA), HA powders (Hydroxyapatite, Sigma-Aldrich Corp., St. Louis, MO, USA), and a compound of β-TCP and HA powders were mixed in the ratio of 40 to 60 were calcined at 600℃ for 2 hours. 25 g of each powder and 4 g of TEP were added to the powder mixture in ethanol for 24 hours by a magnetic stirrer. The slurry was completed by adding 4 g of PVB, and then stirring for 24 hours. The pretreated zirconia scaffolds were dipped in the slurry and residual slurry was removed by air gun. The coated scaffolds were dried at 60℃ for 10 minutes. After this procedure was repeated twice, the coated scaffolds were dried at room temperature for 24 hours. The coated scaffolds were heat-treated at a heating ratio of 3℃/min to 1200℃. After sustaining a temperature of 1200℃ for 2 hours for the purpose of solidification, the coated zirconia scaffolds were slowly cooled.
All samples were ultrasonically cleaned using an ethanol for 10 minutes, washed in running water and finally rinsed in distilled water and dried. All specimens were packed, sealed and sterilized by a gamma radiation (Fig. 1).
The titanium disks, zirconia disks and coated zirconia scaffolds were evaluated by using scanning electron microscope (MIRA LMH, TESCAN, Brno, Czech Republic) and energy dispersive x-ray spectrometer (XFlash Detector 5010, Brunker, Fitchburg, WI, USA).
The crystallography of the specimens was analyzed by x-ray diffractometry (XRD) in an x-ray diffractometer (Minifelx600, Rigaku, Tokyo, Japan). The range of 2θ was from 10° to 70° with a scanning rate of 1°/min.
The coated zirconia scaffolds were immersed in a 10 mL 0.03 M tris-buffer solution (tris(hydroxyl)methyl-aminomethane with hydrochloric acid, T&I Co., Gangwon, Korea) at pH 7.0. These buffer solutions were kept to 37℃ by placing them in a water bath (Wisebath, Daihan Scientific Co. Ltd., Seoul, Korea) for 4 weeks. The calcium and phosphorus ion concentration of buffer solutions were measured by an inductively coupled plasma spectrometer (ICP) (iCAP6300, Thermo scientific, Waltham, MA, USA).
MC3T3-E1 preosteoblastic cells (ATCC cell bank, Manassas, VA, USA) were cultured in normal media containing Dulbecco's modified eagle medium high glucose (DMEM)(Gibco, Grand Island, NJ, USA) supplemented with 10% heat-inactivated fetal bovine serum (FBS) (CELLect GOLD, MP Biomedicals, Irvine, CA, USA), 100 U/mL of penicillin and 10 µg/mL of streptomycin, and maintained at 37℃ in an atmosphere of 5% CO2. The cell culture media was replaced every other day. The attachment and proliferation of MC3T3-E1 cells was determined trypsinizing and cell counting by using a hemocytometer. The MC3T3-E1 cell was seeded onto the disk and scaffold at a density of 1 × 105 cells/mL and cultured for 4 hour and 5 days.
The osteoblastic differentiation of MC3T3-E1 cell was evaluated by a reverse transcriptase-polymerase chain reaction (RT-PCR) analysis of type I collagen (Col1a1), osterix, osteocalcin(OCN), alkaline phosphatase (ALP), Runx2 and β-actin. The cells were plated at a density of 1 × 105 cells/mL on disks and scaffolds. The cells were incubated for 1, 2, 3 and 7 days. The total RNA was isolated from the cultures using the TRIzol reagent (Invitrogen, Carlsbad, CA, USA) according to the manufacturer's instructions. RT-PCR was performed using 1 µg of the total RNA. The total RNA was used for cDNA synthesis by reverse transcriptase with cDNA synthesis kit (Gibco, Grand Island, NJ, USA). The primer sequences were listed on Table 1. Detection of the PCR amplicons was operated by size fractionation by 1% (w/v) agarose gel electrophoresis.
Surfaces were analyzed using scanning electron microscope (SEM) to determine their cellular morphology. MC3T3-E1 cell suspension (3 × 104 cells/mL) was seeded to disks and scaffolds and samples were observed after 3 and 7 days.
After Levene's test was performed to confirm equal variance assumption, an analysis of variance (ANOVA) was conducted to assess the statistical significance of the differences, followed by Scheffé's multiple comparison test, which was done to assess the presence of significant differences (IBM-SPSS Statistics 21, IBM-SPSS Inc., Chicago, IL, USA). P-values <.05 were considered to be significant.
SEM images of titanium disk showed concentric micro-groove patterns form machining, but there was no crystalline structure. Similarly, SEM images of zirconia disk showed irregular micro-groove patterns from grinding before sintering. SEM images of zirconia scaffold showed interconnected pores within the scaffold structure. The pore sizes of scaffolds were approximately 200-500 µm. Coated zirconia scaffolds were partially obturated by coating materials. The crystalline structure of coating material appeared at high magnification. The crystalline structure of HA appeared to have a smaller size than β-TCP (Fig. 2).
Energy dispersive x-ray spectrometer (EDX) profiles were obtained. Titanium, oxygen and platinum were detected at titanium disk. Other elements were omitted because there were only trace elements in error range. Spectrum of zirconia disk and zirconia scaffold showed not only zirconium but also yttrium. From the EDX profiles, coating materials were detected on the zirconia scaffold with coating. Ca/P ratio of zirconia scaffold with β-TCP coating was 1.53. And Ca/P ratio of zirconia scaffold with HA was 1.68. BCP had two types of crystals. The results of EDX confirmed that one was crystal of β-TCP and the other was crystal of HA (Table 2).
XRD patterns of zirconia scaffold with β-TCP, HA and BCP coating were obtained (Fig. 3). The diffraction peaks from the coating varied depending on coating materials. The peaks observed at 27.8°, 31° and 34.4° were attributed to the β-TCP cultured in static circumstance. The peaks at 25.8°, 31.7°, 39.1° and 46.7° were caused by HA. The peaks of zirconia were observed on all groups.
The amount of dissolved Ca2+ ions in the solution was different depending on the coating materials. β-TCP coated zirconia scaffold possessed the largest amount of dissolved Ca2+ ions. Also, more Ca2+ ion was observed on the BCP coated zirconia scaffold than on the HA coated zirconia scaffold. The differences were statistically significant (P<.05).The Ca/P ratio in the solution was different depending on the coating materials (Table 3).
The attachment of MC3T3-E1 cells was measured by using cell counting. After 4 hours of cell seeding, there was no significant difference in all groups. MC3T3-E1 cell proliferation was evaluated by using cell counting after 5 days in culture. Results indicated cell density increased substantially. The cell proliferation of titanium disk appeared significantly lower in comparison with other groups (P<.05). The cell density on zirconia scaffolds with or without coating showed no significant difference, but was significantly higher than on the zirconia disk (P<.05)(Fig. 4).
After incubation of osteoblastic cells for 1 day, the mRNA expression of type I collagen, Runx2, osterix on all groups showed similar activity. The level of ALP mRNA expression increased at 2 days on zirconia disk while it increased at 3 days on the titanium disk. mRNA expression of ALP on zirconia scaffold based group were observed at 1 day. The mRNA level of osteocalcin was higher on non-coated zirconia scaffold compared with the other groups at 7 days (Fig. 5).
After 3 days of cell culture, the MC3T3-E1 cells on the titanium disks were observed flat. SEM images of zirconia disk showed that cells were convex in comparison to these on the titanium disks. Cells on non-coated zirconia scaffold were larger than on other samples. Cells on coated zirconia scaffolds had spindle shape morphology when compared with other samples, but did not have well-developed filopodias (Fig. 6).
After 7 days of cell culturing, a number of cells on the titanium disk were showed flat and spread out. The cells on the zirconia disk were similar to these on the titanium disk, but the number of cells was more than on titanium disk. The cells on non-coated zirconia scaffold were large and had long filopodias. MC3T3-E1 cells on coated zirconia scaffolds had several short filopodias (Fig. 7).
Since crystalline bone screws were first made of aluminum oxide in 1968, many ceramic implants have been commercialized.22,23 Various kinds of ceramics have been used for osseointegration of implants but have some limitation due to their physical properties.24 On the other hand, yttriumoxide partially stabilized zirconia (Y-PSZ) or yttrium-tetragonal zirconia polycrystals (Y-TZP) can be used as a biomaterial with good biocompatibility and high fracture strength. Recently, titanium has been used as a non-absorbable bone graft material.25 It is used for bone grafting during implant placement as well as recovering bone volume for the treatment of peri-implantitis, because it can maintain the stable volume of bone.26
The advantage of zirconia over titanium is that it is a bioinert material with a non-metallic color and good biocompatibility.27 As with titanium, zirconia performs better osseointegration in comparison to other materials, because it does not interfere with the growth of osteoblasts.18
In this study, cells cultured on the titanium disks showed similar initial adhesion properties to the zirconia disks. However, the zirconia disks had a significantly greater positive effect on the proliferation of osteoblasts. Furthermore, the gene expression analysis of the MC3T3-E1 cells showed that the differentiation potency of the cells cultured on the zirconia disks was better than that on the titanium disks. Many studies had shown that zirconia was better on osseointegration than titanium. Sollazzo et al.20 observed that zirconia had specific biological effects in comparison to titanium. Langhoff et al.28 reported that bone to implant contact of zirconia was significantly greater than that of titanium.
The surface treatment of titanium is relatively a simple process, which makes the material popular. But in the case of zirconia, the surface treatment is rarely used because the surface treatment is difficult.
The sintering process is the most common method for fabricating porous scaffolds.21 For the growth of osteoblast, porous surfaces is critical, because cells are attached to the pore and can spread through interconnected pore. Therefore, the activity of osteoblast is better on porous surfaces than on simple rough surface. The pore size is also important and the optimal pore size for osteoblast activity is 100-400 µm.29 In this study, the SEM micrographs showed that the pore size of the zirconia scaffolds was 200 500 µm. The cell proliferation of the zirconia disks was significantly lower than that of the zirconia scaffolds.
As one of the methods for fabricating porous zirconia scaffolds, zirconia powders are mixed with the substances that vaporize relatively at low temperature. After compression molding of mixture, sintering produces pore area with burning out. This method is very simple but it is impossible to make well-formed interconnecting pores.30 The method of coating open cell sponge foams after sintering was difficult to perform due to sintering temperature and coating process. However, it could make great interconnecting pores.
There are 2 steps in the process of osseointegration at the cellular level. The first process is the attachment and spreading of preosteoblastic cell. It is the process of quantitative growth. The second process is a differentiation of cells, which is the process of qualitative growth. During the initial stage of cell attachment, the superficial dimension is an important factor. In this study, a porous scaffold was used to increase the superficial dimension.
The SEM micrographs showed the different coating state according to the coating materials. The crystals of β-TCP were larger and looser than those of HA. The crystalline of HA was small but closely arranged. The surfaces were coated with small crystals of HA, and then β-TCP in BCP coating. Ca2+ ions in the solution were dependent on the coating materials. The β-TCP coating had the largest amount of dissolved Ca2+ ions due to its higher solubility than HA. The second largest amounts were found in the BCP and HA coatings. This was considered to be the microporosity of the coating layer. The Ca/P ratio in the solution was different depending on the coating materials. Because the zirconia scaffold is an inert material, it showed the Ca/P ratio of the coating materials. The Ca/P ratios of the zirconia scaffold in the solution of β-TCP, HA and BCP coating were 1.52, 1.68 and 1.63 respectively, which were similar to those of β-TCP, HA and BCP (1.5, 1.67 and 1.608). An energy dispersive spectrograph also showed similar results. According to EDX, the Ca/P ratios of β-TCP and HA were 1.53 and 1.68, and consistent with those of ICP analysis. This means that the coating procedures were properly performed as planned. The Ca/P ratio in the solution of BCP coating was not statistically significant. As in the SEM micrograph of BCP coating, the crystal of HA was greater than that of β-TCP.
A MC3T3-E1 mouse pre-osteoblastic cell was used for this study due to its high level of differentiation and its ability to confirm bone markers such as type I collagen, osterix, osteocalcin, ALP and Runx2, making it an excellent model for cell differentiation.31,32 Type I collagen is a major extracellular matrix of fibroblasts.33 Osterix is an essential transcription factor for osteoblast differentiation. The function of osterix is regulated by Runx2. Osteocalcin is an osteoblast-specific protein and is secreted by osteoblasts to play a role in the metabolic regulation of calcium ion.34 Runx2 is the best known as a key regulator of osteoblast marker genes. Runx2 can also directly stimulate the transcription of osteoblast-related genes such as those encoding osteocalcin (OCN), type I collagen, osteopontin (OPN).35 The mRNA levels of β-actin, commonly called housekeeping gene, served as a control. Relative densitometric data were normalized to the corresponding β-actin mRNA levels.
This study investigated the osteogenic response to the coating material. The coating material was confirmed by SEM, EDX and XRD. The coated scaffold was partially obturated as compared to the non-coated zirconia scaffold in SEM micrographs. During the coating process, the clogging interconnecting pore was avoided by using an air gun. However, it was difficult to remove all of the coating materials at the bottom. Because the solvent of the slurry of coating materials was evaporated quickly by using an air gun, it was considered that coating materials remained interporous. There was no difference in cell proliferation between non-coated scaffolds and coated scaffolds. However, there was a significant difference in cell differentiation. No difference in cell attachment and proliferation was observed despite partial obturation of interconnecting pores, but the activity of cell differentiation was greater in non-coated zirconia scaffolds with interconnecting pores. After 7 days in the cultures, the mRNA expression of osteocalcin was distinctly detected only in the non-coated zirconia scaffolds. Therefore, the differentiation of osteoblast cells was influenced by microstructure rather than coating material.36
It is concluded that the activity of osteoblast in zirconia was higher than in titanium. The non-coated zirconia scaffold had clear inter-connecting pore. β-TCP coated scaffold was expected to be the best in terms of osteogenic activity. The results of this study showed that the non-coated zirconia scaffold was superior. It was found from the results that the activity of osteoblasts was more affected by microstructure than coating material. The limitation of this study was that it was at the cellular level for a short term, and that the coating material obturated interconnecting pore partially.
For the commercialization of a zirconia bone graft, a proper coating method should be used in order to ensure optimal osteogenic process while maintaining interconnecting pores. In addition, there is a need for further in vivo studies to make improvement in material properties.
Based on the results of this study, the osteoblast cell activity on zirconia was higher than on titanium. The interconnecting pores of zirconia scaffolds showed enhanced proliferation and cell differentiation. Further research is required to investigate the influence of coating materials and microstructures such as interconnecting pore on differentiation of osteoblast in a tightly controlled environment.
Figures and Tables
Fig. 1
Samples for cell culture. Machined titanium disk (A), zirconia disk (B), zirconia scaffold (C), zirconia scaffold with β-TCP coating (D), zirconia scaffold with HA coating (E) and zirconia scaffold with BCP coating (F).
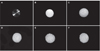
Fig. 2
Scanning electron micrographs. Titanium (A: × 100, B: × 2,000), zirconia (C: × 100, D: × 2,000), non-coated zirconia scaffold (E: × 100, F: × 2,000), zirconia scaffold with β-TCP coating (G: × 100, H: × 5,000), zirconia scaffold with HA coating (I: × 100, J: × 5,000), and zirconia scaffold with BCP coating (K: × 100, L: × 5,000).
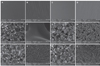
Fig. 3
XRD patterns of different coating materials on zirconia scaffold. Zirconia scaffold with β-TCP coating (A), zirconia scaffold with HA coating (B) and zirconia scaffold with BCP coating (C).
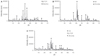
Fig. 4
Comparison of cell ccounts according to samples after 5 days of cell seeding.
*denotes difference significant at 0.05 value.
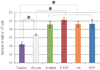
Fig. 5
RT-PCR analysis for gene expression of type I collagen, osterix, osteocalcin, alkaline phosphatase, Runx2 and β-actin. Titanium disk (A), zirconia disk (B), non-coated zirconia scaffold (C), zirconia scaffold with β-TCP coating (D), zirconia scaffold with HA coating (E) and zirconia scaffold with BCP coating (F).
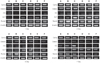
Fig. 6
Scanning electron micrographs of cultured MC3T3-E1 cells after 3 days (magnification × 1,000). Titanium disk (A), zirconia disk (B), non-coated zirconia scaffold (C), zirconia scaffold with β-TCP coating (D), zirconia scaffold with HA coating (E) and zirconia scaffold with BCP coating (F).
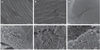
Fig. 7
Scanning electron micrographs of cultured MC3T3-E1 cells after 7 days (disks:magnification × 300, scaffolds: magnification × 1,000). Titanium disk (A), zirconia disk (B), non-coated zirconia scaffold (C), zirconia scaffold with β-TCP coating (D), zirconia scaffold with HA coating (E) and zirconia scaffold with BCP coating (F).
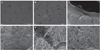
Table 1
Gene-specific primer sequences of MC3T3-E1 cells used in RT-PCR
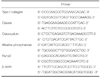
Table 2
Atomic percentage of the elements obtained by energy dispersive x-ray spectrometer
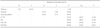
References
1. Hench LL, Polak JM. Third-generation biomedical materials. Science. 2002; 295:1014–1017.
2. Fransson C, Lekholm U, Jemt T, Berglundh T. Prevalence of subjects with progressive bone loss at implants. Clin Oral Implants Res. 2005; 16:440–446.
3. Klinge B. Peri-implant marginal bone loss: an academic controversy or a clinical challenge? Eur J Oral Implantol. 2012; 5:S13–S19.
4. Kim YK, Kim SG, Yun PY, Yeo IS, Jin SC, Oh JS, Kim HJ, Yu SK, Lee SY, Kim JS, Um IW, Jeong MA, Kim GW. Autogenous teeth used for bone grafting: a comparison with traditional grafting materials. Oral Surg Oral Med Oral Pathol Oral Radiol. 2014; 117:e39–e45.
5. Bae JH, Kim YK, Kim SG, Yun PY, Kim JS. Sinus bone graft using new alloplastic bone graft material (Osteon)-II: clinical evaluation. Oral Surg Oral Med Oral Pathol Oral Radiol Endod. 2010; 109:e14–e20.
6. Dalkýz M, Ozcan A, Yapar M, Gökay N, Yüncü M. Evaluation of the effects of different biomaterials on bone defects. Implant Dent. 2000; 9:226–235.
7. Carotenuto G, Spagnuolo G, Ambrosio L, Nicolais L. Macroporous hydroxyapatite as alloplastic material for dental applications. J Mater Sci Mater Med. 1999; 10:671–676.
8. Lu J, Descamps M, Dejou J, Koubi G, Hardouin P, Lemaitre J, Proust JP. The biodegradation mechanism of calcium phosphate biomaterials in bone. J Biomed Mater Res. 2002; 63:408–412.
9. Chao SY, Poon CK. Histologic study of tissue response to implanted hydroxylapatite in two patients. J Oral Maxillofac Surg. 1987; 45:359–362.
10. Frame JW, Browne RM, Brady CL. Hydroxyapatite as a bone substitute in the jaws. Biomaterials. 1981; 2:19–22.
11. Daculsi G, LeGeros RZ, Nery E, Lynch K, Kerebel B. Transformation of biphasic calcium phosphate ceramics in vivo: ultrastructural and physicochemical characterization. J Biomed Mater Res. 1989; 23:883–894.
12. Nery EB, LeGeros RZ, Lynch KL, Lee K. Tissue response to biphasic calcium phosphate ceramic with different ratios of HA/beta TCP in periodontal osseous defects. J Periodontol. 1992; 63:729–735.
13. Gauthier O, Bouler JM, Aguado E, Pilet P, Daculsi G. Macroporous biphasic calcium phosphate ceramics: influence of macropore diameter and macroporosity percentage on bone ingrowth. Biomaterials. 1998; 19:133–139.
14. Daculsi G, Passuti N, Martin S, Deudon C, Legeros RZ, Raher S. Macroporous calcium phosphate ceramic for long bone surgery in humans and dogs. Clinical and histological study. J Biomed Mater Res. 1990; 24:379–396.
15. Jiang G, Shi D. Coating of hydroxyapatite on highly porous Al2O3 substrate for bone substitutes. J Biomed Mater Res. 1998; 43:77–81.
16. Pae A, Lee H, Kim HS, Baik J, Woo YH. Cellular attachment and gene expression of osteoblast-like cells on zirconia ceramic surfaces. J Korean Acad Prosthodont. 2008; 46:227–237.
17. Guazzato M, Quach L, Albakry M, Swain MV. Influence of surface and heat treatments on the flexural strength of Y-TZP dental ceramic. J Dent. 2005; 33:9–18.
18. Piconi C, Maccauro G. Zirconia as a ceramic biomaterial. Biomaterials. 1999; 20:1–25.
19. Piconi C, Burger W, Richter HG, Cittadini A, Maccauro G, Covacci V, Bruzzese N, Ricci GA, Marmo E. Y-TZP ceramics for artificial joint replacements. Biomaterials. 1998; 19:1489–1494.
20. Sollazzo V, Pezzetti F, Scarano A, Piattelli A, Bignozzi CA, Massari L, Brunelli G, Carinci F. Zirconium oxide coating improves implant osseointegration in vivo. Dent Mater. 2008; 24:357–361.
21. Schultze-Mosgau S, Schliephake H, Radespiel-Tröger M, Neukam FW. Osseointegration of endodontic endosseous cones: zirconium oxide vs titanium. Oral Surg Oral Med Oral Pathol Oral Radiol Endod. 2000; 89:91–98.
22. Albrektsson T, Zarb G, Worthington P, Eriksson AR. The long-term efficacy of currently used dental implants: a review and proposed criteria of success. Int J Oral Maxillofac Implants. 1986; 1:11–25.
23. Andreiotelli M, Wenz HJ, Kohal RJ. Are ceramic implants a viable alternative to titanium implants? A systematic literature review. Clin Oral Implants Res. 2009; 20:32–47.
24. McKinney RV Jr, Steflik DE, Koth DL. The biologic response to the single-crystal sapphire endosteal dental implant: scanning electron microscopic observations. J Prosthet Dent. 1984; 51:372–379.
25. Vagkopoulou T, Koutayas SO, Koidis P, Strub JR. Zirconia in dentistry: Part 1. Discovering the nature of an upcoming bioceramic. Eur J Esthet Dent. 2009; 4:130–151.
26. Wohlfahrt JC, Lyngstadaas SP, Rønold HJ, Saxegaard E, Ellingsen JE, Karlsson S, Aass AM. Porous titanium granules in the surgical treatment of peri-implant osseous defects: a randomized clinical trial. Int J Oral Maxillofac Implants. 2012; 27:401–410.
27. Steinemann SG. Titanium--the material of choice? Periodontol 2000. 1998; 17:7–21.
28. Langhoff JD, Voelter K, Scharnweber D, Schnabelrauch M, Schlottig F, Hefti T, Kalchofner K, Nuss K, von Rechenberg B. Comparison of chemically and pharmaceutically modified titanium and zirconia implant surfaces in dentistry: a study in sheep. Int J Oral Maxillofac Surg. 2008; 37:1125–1132.
29. Itälä AI, Ylänen HO, Ekholm C, Karlsson KH, Aro HT. Pore diameter of more than 100 microm is not requisite for bone ingrowth in rabbits. J Biomed Mater Res. 2001; 58:679–683.
30. Aboushelib MN, Salem NA, Taleb AL, El Moniem NM. Influence of surface nano-roughness on osseointegration of zirconia implants in rabbit femur heads using selective infiltration etching technique. J Oral Implantol. 2013; 39:583–590.
31. Sudo H, Kodama HA, Amagai Y, Yamamoto S, Kasai S. In vitro differentiation and calcification in a new clonal osteogenic cell line derived from newborn mouse calvaria. J Cell Biol. 1983; 96:191–198.
32. Wang D, Christensen K, Chawla K, Xiao G, Krebsbach PH, Franceschi RT. Isolation and characterization of MC3T3-E1 preosteoblast subclones with distinct in vitro and in vivo differentiation/mineralization potential. J Bone Miner Res. 1999; 14:893–903.
33. Pae A, Lee H, Kim HS, Kwon YD, Woo YH. Attachment and growth behaviour of human gingival fibroblasts on titanium and zirconia ceramic surfaces. Biomed Mater. 2009; 4:025005.
34. Tai G, Christodoulou I, Bishop AE, Polak JM. Use of green fluorescent fusion protein to track activation of the transcription factor osterix during early osteoblast differentiation. Biochem Biophys Res Commun. 2005; 333:1116–1122.
35. Franceschi RT, Xiao G. Regulation of the osteoblast-specific transcription factor, Runx2: responsiveness to multiple signal transduction pathways. J Cell Biochem. 2003; 88:446–454.
36. Barrère F, van der Valk CM, Dalmeijer RA, Meijer G, van Blitterswijk CA, de Groot K, Layrolle P. Osteogenecity of octacalcium phosphate coatings applied on porous metal implants. J Biomed Mater Res A. 2003; 66:779–788.