Abstract
Adult hippocampal dentate granule neurons are generated from neural stem cells (NSCs) in the mammalian brain, and the fate specification of adult NSCs is precisely controlled by the local niches and environment, such as the subventricular zone (SVZ), dentate gyrus (DG), and Toll-like receptors (TLRs). Epigallocatechin-3-gallate (EGCG) is the main polyphenolic flavonoid in green tea that has neuroprotective activities, but there is no clear understanding of the role of EGCG in adult neurogenesis in the DG after neuroinflammation. Here, we investigate the effect and the mechanism of EGCG on adult neurogenesis impaired by lipopolysaccharides (LPS). LPS-induced neuroinflammation inhibited adult neurogenesis by suppressing the proliferation and differentiation of neural stem cells in the DG, which was indicated by the decreased number of Bromodeoxyuridine (BrdU)-, Doublecortin (DCX)- and Neuronal Nuclei (NeuN)-positive cells. In addition, microglia were recruited with activatingTLR4-NF-κB signaling in the adult hippocampus by LPS injection. Treating LPS-injured mice with EGCG restored the proliferation and differentiation of NSCs in the DG, which were decreased by LPS, and EGCG treatment also ameliorated the apoptosis of NSCs. Moreover, pro-inflammatory cytokine production induced by LPS was attenuated by EGCG treatment through modulating the TLR4-NF-κB pathway. These results illustrate that EGCG has a beneficial effect on impaired adult neurogenesis caused by LPSinduced neuroinflammation, and it may be applicable as a therapeutic agent against neurodegenerative disorders caused by inflammation.
Neuroinflammation with microglial activation plays a role in neurodegenerative diseases, such as Alzheimer's disease and multiple sclerosis [1], and microglia involved in the inflammation of the central nervous system have neuroprotective effects [234]. Recent in vivo and in vitro studies have suggested that microglia are essential in neural development, synapse formation, and neuroinflammation involved in the impairment of adult neurogenesis [567]. This evidence was supported by the Gram-negative bacterial immune stimulation lipopolysaccharide (LPS)-induced neuroinflammatory model, which showed that LPS-induced activation of the Toll-like receptor 4 (TLR4) in microglia led to the production of the pro-inflammatory cytokines, IL-1β, IL-6, and TNF-α through the MAPK and NFκB pathways in BV2 cell lines [89]. In addition to the immune response by activated microglia, the neuroprotective cytokines, IL-4 and IL-25, are also produced [1011]. Moreover, other studies showed that adult neurogenesis in the hippocampus was affected through the TLR and NF-κB pathway in microglia [1213].
Adult neurogenesis is a process in which new neurons are continuously generated throughout adult life from the neural stem cells (NSCs) in the mammalian brain. Small populations of neurons continue to be born in the adult subventricular zone, the olfactory system, and the DG of the hippocampus. The neurogenic niches are important for the NSCs to have self-renewal and multipotent properties that can give rise to neurons, astrocytes, and oligodendrocytes [141516]. The neuronal fate specification (neurogenesis) and glial fate specification (gliogenesis) of the NSCs is determined by cellular communication within the microenvironment [17]. The newborn neurons are critical to cognitive functions including learning and memory. For instance, physical activity and an enriched environment, which are associated with improved memory function and synaptic plasticity, enhance adult neurogenesis in the DG [1819]. Furthermore, adult neurogenesis in the DG declines with aging and neurodegenerative diseases, which in turn causes cognitive deficits [202122].
Green tea has been shown to function as an anticancer reagent that inhibits the development and progression of skin, lung, mammary gland, and gastrointestinal tract tumors in animal models [2324]. Among the various polyphenols in green tea including epigallocatechin-3-gallate (EGCG), (–)-epigallocatechin, (–)-epicatechin, (+)-gallocatechin, and other catechins, EGCG is the most abundant and the most biologically active component [252627]. However, the recovery effects of EGCG on adult neurogenesis following neuroinflammation at the DG in the hippocampus are still unknown. In the present study, we investigate the effect of EGCG on impaired adult neurogenesis in a mouse model of LPS-induced neuroinflammation.
Here, we present the effects of EGCG on impaired adult hippocampal neurogenesis in vivo caused by neuroinflammation. We induced inf lammation by administering LPS in a mouse model and explored the positive effects of EGCG in NSC proliferation and neural differentiation using an immunohistochemical approach to detect bromodeoxyuridine (BrdU, an S-phase marker of proliferating cells), doublecortin (DCX, a marker of immature newborn neurons), neuronal nuclei (NeuN, a marker of mature neurons), ionized calcium binding adapter molecule 1 (Iba-1, a marker of microglia), and the levels of cytokines.
7-week-old male C57BL/6 mice were purchased from Daehan Biolink (Chungbuk, Korea). The animals were kept under standard conditions of 22℃ and a humidity of 55% with a 12 hour light-12 hour dark cycle, and allowed free access to food and water. Mice were divided into the following three groups: saline-treated group (sham control), saline-treated with lipopolysaccharide (LPS, E.coli, serotype 055:B5, St. Louis, MO, USA) intracerebroventricular (I.C.V.) injection group, and epigallocatechin-3-gallate (EGCG)-treated with LPS I.C.V. injection group (n=5 mice/each group). Supplementary Figure 1 demonstrated the schematic experimental design of time courses with LPS, EGCG and BrdU injection followed by sampling the brain for immunohistochemistry. All experiments were approved by the Animal Care and Use Committee of Chonnam National University. All chemicals used in the experiments were supplied by Sigma (St. Louis, MO, USA).
Experimental mice were anesthetized with zoletil (VIRBAC, Milperra, Australia) (25 mg/kg) and Rompun (Bayer HealthCare, Mississauga, Canada) (10 mg/kg). LPS for a single unilateral stereotaxic injection was prepared in phosphate-buffered saline (PBS, pH 7.4) at a final concentration of 1.0 mg/ml and was injected into the brain (0.6 mm caudal to the bregma, 1.5 mm to the right of the bregma, 2.0 mm ventral to the bregma). Mice were injected with vehicle (sterile saline) or LPS (3 µg in 3 µl sterile saline) following brain surgery at a dose of 1 µg/min.
EGCG (0.5 mg/kg) prepared in physiological saline (PBS containing 0.9% NaCl) was injected intraperitoneally (i.p.) for three times with 6 hours interval at 3 hours after recovered from anesthesia for LPS-injection.
BrdU (Sigma, St. Louis, MO, USA) was dissolved in physiological saline and injected i.p. (50 mg/kg). To examine the proliferation of NSCs in the DG, BrdU was injected into the animals once, 3 hours prior to sacrifice. To study the survival rate of newborn cells generated from NSCs and the differentiation rate of NSCs, BrdU was injected once daily for 5 consecutive days and the mice were sacrificed on 28th day after the final BrdU injection.
Animals were perfused transcardially with 0.1 M PBS, followed by fresh cold 4% paraformaldehyde (PFA) in 0.1 M PBS. The brains were removed and fixed overnight in 4% PFA in 0.1 M PBS at 4℃, and then washed for 6 hours in PBS at 4℃. Sucrose-saturated brains were then embedded in freezing media (O.C.T compound, Leica Biosystems, Richmond, USA), frozen in chilled isopentane (–25℃), and stored at –80℃ until sectioning. Brains were cryocut coronally at a thickness of 40 mm using a Cryostat (Model CM3050; Leica Microsystems, Richmond, USA) and stored in cryoprotectant solution (25% ethylene glycol, 25% glycerol, and 0.05 M sodium phosphate buffer, Na-PB) at –20℃ until immunohistochemical (IHC) processing.
Sections were washed in Na-PB and mounted on charged slide glasses for IHC. The sections immunostained for BrdU were pretreated with 2 N HCl for 30 minutes at 37℃ and neutralized with PBS before incubation with primary antibodies. Sections were incubated for 60 minutes with 5% normal horse serum in 0.4% Triton X-100 in PBS (PBST). The sections were incubated overnight at 4℃ with primary antibodies in the same buffer solution. Primary antibodies were used at the following concentrations: rat anti-BrdU (1:200; Abcam, Cambridge, UK), goat anti-doublecortin (DCX, 1:100; Cell Signaling, Danvers, MA), mouse anti-neuronal nuclear antigen (NeuN, 1:100; Chemicon, Temecula, CA), and rabbit anti-ionized calcium-binding adapter molecule 1 (Iba-1, 1:100; Wako Chemicals USA, Inc., Richmond, VA). Sections were washed three times with PBST for 10 minutes at room temperature and blocked in PBST containing 5% horse serum for 30 minutes. Sections were then incubated for 2 hours with secondary antibodies conjugated to FITC (Jackson Immuno-Research, West Grove, PA) or CY3 (Jackson Immuno-Research, West Grove, PA). The sections were washed three times with PBST, and stained with 10 mg/ml 4'6'-diamidino-2-phenylindole (DAPI) (Sigma-Aldrich, St. Louis, USA) for 30 minutes before mounted.
IHC images were acquired with a confocal microscope (Zeiss, Thornwood, NY) equipped with an argon/krypton laser (488 nm), two helium/neon lasers (543 and 633 nm), and a coherent laser (Santa Clara, CA), using the 20x objective lens. Images were analyzed with the Image J (Ver.1.4, NIH, USA). Cells were counted in a defined frame size of 20~50 with 35 µm×35 µm in every selected section. Colocalizations were investigated by performing z-stack acquisitions and three-dimensional reconstructions with the LSM software (Zeiss, Thornwood, NY). Adobe Photoshop (Version CS6, Adobe Systems, San Jose, CA) was used to adjust the contrast and brightness.
Brain was homogenized in RIPA buffer (150 mM NaCl, 1.0% IGEPAL® CA-630, 0.5% sodium deoxycholate and 0.1% SDS in 50 mM Tris, Sigma, St. Louis, MO, USA). Proteins were separated by SDS-PAGE and transferred to a nitrocellulose membrane. The membranes were incubated with primary anti-TLR4, anti-RelA, anti-pRelA, and anti-Actin antibodies (Cell Signaling, Danvers, MA or Abcam, Cambridge, UK). The expression levels of protein were visualized and analyzed with Fusion FX (Vilber Lourmat, Eberhardzell, Germany).
The levels of IL-1β, IL-6, and TNF-α in the DG were measured using a commercial ELISA kit (R&D Systems, Minneapolis, MN, USA) as per the manufacturer's instructions, and using quantitative real-time PCR (Bio-Rad Lab. Inc., CA, USA). In detail, the total RNAs were prepared using Trizol Reagent (Gibco BRL) and reverse transcription was performed with a RT system containing Moloney Murine Leukemia Virus reverse transcriptase (Promega, Madison, WI) in accordance with the manufacturer's instructions. PCR was performed in a Palm-Cycler thermocycler (Corbett Life Science, Sydney, Australia) and the product was resolved in a 1.2% agarose gel. Real time amplification of cDNA was conducted in a Rotor-Gene 3000 System (Corbett Research, Morklake, Australia) using the SYBR Green PCR Master Mix Reagent Kit (Qiagen, Valencia, CA). The PCR conditions were as follows: incubation for 5 minutes at 95℃, followed by 30 cycles of denaturation for 15 seconds at 95℃, annealing for 15 seconds at 62℃ and extension for 15 seconds at 72℃. The primers were as follows: mouse IL-1β; 5'-AGG AGA ACC AAG CAA CGA CA-3' and 5'-CTT GGG ATC CAC ACT CTC CAG-3', mouse IL-6; 5'-GCC TTC TTG GGA CTG ATG CT-3' and 5'-GCC TTC TTG GGA CTG ATG CT-3', mouse TNF-α; 5'-ATG GCC TCC CTC TCA TCA GT-3' and 5'-CTT GGT GGT TTG CTA CGA CG-3' and β-actin; 5'-GAT CTG GCA CCA CAC CTT CT-3' and 5'-GGG GTG TTG AAG GTC TCA AA-3'. The relative levels of mRNA were calculated using the standard curve generated from the cDNA dilutions. The mean cycle threshold (Ct) values from quadruplicate measurements were employed in the calculation of gene expression, with normalization to β-actin as an internal control. Calculation of the relative levels of gene expression was performed using Corbett Robotics Rotor-Gene software (Rotor-Gene 6 version 6.1, Build 90).
A complete series of 1 in 10 sections of the DG was analyzed. For each experiment, 9 sections per mouse were selected for analysis. The immunolabeled cells were counted and multiplied by 10 to obtain the total number of labeled cells throughout the DG. The data are expressed as the mean±SEM. Statistical analyses were performed using a one-way analysis of variance (ANOVA) followed by a Student-Newman-Keuls test for multiple comparisons. Results were considered statistically significant when the p value was less than 0.05.
To study the effect of EGCG in the DG related to neurogenesis, the proliferation of adult NSCs in the DG was initially examined in an LPS-induced neuroinf lammation mouse model by administering LPS (3 µg/animal with 1 mg/ml stock) via intracerebroventricular (I.C.V.) injection (n=5 each). The number of BrdU (S-phase marker)-positive cells were compared at 3 hours following a single BrdU injection (i.p. 50 mg/kg), which indicates proliferating cells, and were found to be significantly decreased at 1 day (0.7066±0.04408×103, 38% of decreased compared to sham control, F (4.4)=1.076, p=0.0002, two-tailed unpaired one-way ANOVA) and day 3 (0.8292±0.03464×103, 29% of decreased compared to sham control, F (4.4)=1.577, p=0.0003, two-tailed unpaired one-way ANOVA) post-LPS injection in mice treated with either vehicle or EGCG compared with the sham controls. However, EGCG significantly improved the number of BrdU-positive cells affected by LPS in the hippocampal DG (day1; 0.8242±0.01520×103, 16% of increased compared with LPS only, F (4.4)=8.406, p=0.0357, day 3; 0.9462±0.03309×103, 14% of increased compared with LPS only, F (4.4)=1.096, p=0.0404, two-tailed unpaired one-way ANOVA) (Fig. 1). These results show that treatment with EGCG recovered NSC proliferation, which was impaired by LPS-induced neuroinflammation.
DCX (immature neuronal marker) is transiently expressed in the soma and dendrites of immature newborn neurons. The newborn NSCs are differentiated into neurons to serve as functional units in the nervous system following their proliferation. Thus, we studied whether EGCG ameliorated the differentiation of adult NSCs after LPS-induced injury by counting the number of BrdU- and DCX double-positive cells and the ratio of BrdU+ DCX+ cells/BrdU+ cells in the hippocampal DG 5 days after injection with BrdU (n=5 each). The number of double positive cells was decreased post LPS injection compared to that of sham controls (1.070±0.06641×103, 32% of decreased compared to sham control, F (4.4)=1.287, p=0.0013), but it was significantly improved after injection with EGCG in the LPS-injured group compared with the LPS-injured group with no treatment (1.398±0.07632×103, 30% of increased compared to LPS only, F (4.4)=1.321, p=0.0118) (Fig. 2A and B). Similarly, the ratio of the total number of BrdU- and DCX-double positive cells to the total number of BrdU-positive cells was also decreased in the LPS-treated group compared with the sham controls (43.60±1.887×103, 11.4% of decreased compared to sham control, F (4.4)=1.017, p=0.0026), but the ratio was recovered in the EGCG-treated LPS-injured group compared with the LPS-injured group with no treatment (50.80±1.985×103, 7.2% of increased compared to LPS only, F (4.4)=1.107, p=0.0302, two-tailed unpaired one-way ANOVA) (Fig. 2A and C). These results suggest that EGCG had a recovery effect on the neuronal differentiation of adult NSCs, which was impaired by LPS in the early stage.
To investigate the effect of EGCG on the maturation of newborn neurons following LPS-induced neuroinflammation, immunohistochemical analysis was performed using antibodies against the mature neuronal marker, NeuN, and BrdU. The results show that the number of cells co-localized with NeuN and BrdU are decreased in the LPS-injured group (0.6280±0.03967×103, 27% of decreased compared to sham control, F (4.4)=1.623, p=0.0021), but the number of these cells are increased in the EGCG-treated LPS-injured group compared with the vehicle-treated LPS-injured group (0.7476±0.02307×103, 19% of increased compared to LPS only, F (4.4)=2.958, p=0.0313) (Fig. 3A and B). Furthermore, the ratio of NeuN/BrdU double-positive cells to the total BrdU-positive cells in the EGCG-treated LPS-injured group was significantly increased compared with that of the vehicle-treated LPS-injured group (52.80±1.158, 5.6% of increased compared to LPS only, F(4.4)=2.791, p=0.0378, two-tailed unpaired one-way ANOVA), which was decreased compared with the sham control group (Fig. 3C). These results support the notion of a beneficial effect of EGCG on mature neuronal differentiation of adult NSCs, which was impaired by LPS-induced neuroinflammation.
Following NSC proliferation in the DG, the newborn NSCs survive and differentiate into neurons. To determine the survival rate of newborn cells derived from NSCs in the DG, the number of BrdU-positive cells was quantified 3 hours and 28 days after the last injection of BrdU, which was injected once a day for 5 consecutive days. The total number of BrdU-positive cells was decreased in the LPS-injured group, and was recovered in the EGCG-treated LPS-injured group up to the level of the sham controls (n=5 each). Subsequently, we quantified the survival rate of newborn cells by counting the total BrdU-positive cells, 28 days after the final of 5 consecutive days of BrdU injections, to BrdU-positive cells, 3 hours after the final of 5 consecutive days of BrdU injections. The rate was decreased in all groups, but the EGCG-treated LPS-injured group showed an improved survival rate of newborn cells compared with the LPS-injured group with no treatment (43.20±0.8602, 3.8% of increased compared to LPS only, F (4.4)=1.162, p=0.0170, two-tailed unpaired one-way ANOVA) (Fig. 4A and C). Further, to examine the effect of EGCG on newborn cell survival related to apoptosis, the number of cleaved caspase 3-positive cells was counted. The total number of cleaved caspase 3-positive cells was significantly decreased in the EGCG-treated LPS-injured group compared with the vehicle-treated LPS-injured group (0.4880±0.01393×102, 12% of decreased compared to LPS only, F (4.4)=1.062, p=0.0109, two-tailed unpaired one-way ANOVA) (Fig. 4B and D). The results show that neuroinflammation decreased the survival of dividing cells, whereas EGCG recovered the survival of dividing cells, which was impaired by LPS-induced neuroinflammation.
To study the effect of EGCG on neuroinflammation in detail, the activity of microglia following LPS I.C.V. injection was quantified by the immunoreactivity of Iba-1, a specific marker of microglia (n=5 each). The number of Iba-1-positive cells in the DG was increased (day 1; 4.876±0.2238×103, 54% of increased compared to sham control, F (4.4)=2.181, p=0.0002, day 3; 4.528±0.2567×103, 39% of increased compared to sham control, F (4.4)=2.917, p=0.0025, two-tailed unpaired one-way ANOVA ) by LPS injection, which was suppressed at day 1 and day 3 of EGCG treatment (day 1; 3.393±0.2497×103, 7% of decreased compared to LPS only, F (4.4)=1.245, p=0.0022, day 3; 3.402±0.1609×103, 4% of decreased compared to LPS only, F (4.4)=2.544, p=0.0059, two-tailed unpaired one-way ANOVA ) (Fig. 5A and B). Since LPS acted as a TLR4 agonist, the expression of TLR4, NF-κB, and cytokines was examined as molecular targets of EGCG in adult neurogenesis impaired by LPS-induced neuroinflammation. The expression of TLR4 and phospho-RelA (activated form) was upregulated in the LPS-injured group compared with the sham controls, but the expression of TLR4 and phospho-RelA was suppressed in the EGCG-treated LPS-injured group compared with the LPS-injured group. Therefore, LPS activated the expression of TLR4 and stimulated NF-κB, but EGCG attenuated the LPS-induced TLR4 and NF-κB (Fig. 5C). In addition, LPS upregulated the production of IL-1β, IL-6, and TNF-α by microglia, however, the increased mRNA and protein levels of cytokines induced by LPS injection were compromised by EGCG up to 53.75%, 46.67%, and 30.71% for IL-1β, IL-6 and TNF-α transcripts, respectively, and up to 72.61%, 47.59% and 59.57% for proteins, respectively, compared with the expression levels of the LPS-injured group (p<0.05) (Fig. 5D~G). The results demonstrate that EGCG suppressed the inflammatory activities of microglia, resulting in the attenuation of proinflammatory cytokine production via the TLR4/NF-κB signaling pathway.
Adult NSCs in the hippocampal DG have the ability to generate self-renewing neural stem cells possessing multipotency [28]. Adult neurogenesis occurs in three discrete stages, proliferation of NSCs, cell survival, and neuronal differentiation, which are affected by various factors such as hormones, growth factors, enriched environment, stress, drugs, and pathological stimulation [2930]. The process in the DG affected by these factors is associated with cognitive functions including learning and memory [31]. Neuroinflammation in the brain following injuries causes neural development-related diseases, which modulate adult hippocampal neurogenesis [3233]. During the inflammatory response following injury to the central nervous system (CNS), microglial cells and a population of glial cells of the CNS as immune cells produce proinflammatory cytokines. In particular, IL-6 and NO affect adult hippocampal neurogenesis, which are involved in the pathogenesis of neurological diseases such as Alzheimer's disease (AD) and Parkinson's disease (PD) [3435].
EGCG, among the catechins, a major subgroup of polyphenolic flavonoids in green tea, is well-known for its anti-carcinogenic effects via the suppression of the proliferation and angiogenesis of cancer cells [36]. Even though the instability of catechins, previous studies were showing that EGCG was effectively delivered over blood brain barrier and was founded physiologically activated forms in the brain [3738]. In addition, it may be used for the prevention and treatment of AD and PD based on the evidence that EGCG promotes adult hippocampal neurogenesis [394041]. Moreover, recent studies have demonstrated that EGCG had a neuroprotective effect through the reduction of neuroinflammation [42434445]. Oxidative stress has been implicated in the pathophysiology of the majority of neurodegenerative diseases, and most of the researches in this field have focused on EGCG as a natural pharmacological compound [46]. However, there have been no studies assessing the effect of EGCG on neurogenesis in the DG of the adult hippocampus impaired by neuroinflammation. Therefore, here, we investigated whether EGCG improves adult neurogenesis via the modulation of neuroinflammation in vivo.
In the present study, the effect of EGCG on the proliferation of adult NSCs in the DG following LPS injection was investigated. Proliferation of adult NSCs in the DG was inhibited by LPS injection, however, the number of BrdU-positive cells indicating the rate of adult NSC proliferation was improved within one day in the EGCG-treated LPS-injured group (Fig. 1A~C). Moreover, the survival rate of newborn cells, represented by the ratio of the total number of BrdU-positive cells 28 days after the final BrdU injection following LPS injection, was amended by EGCG treatment (Fig. 2A~C). These results suggest that EGCG rescued the proliferation and survival of adult NSCs in the DG impaired by LPS-induced neuroinflammation, which is consistent with that of previous reports showing the effective role of EGCG in increasing cell proliferation in mouse hippocampal DG and neural progenitor cell proliferation during adult hippocampal neurogenesis [4748].
To investigate the effect of EGCG on immature neuronal differentiation in the DG following LPS-induced neuroinflammation, immunohistochemical analysis was performed using a marker of immature neural differentiation, DCX, and BrdU, 5 days after BrdU injection following LPS with or without EGCG injection. The number of double positive cells (DCX+ and BrdU+) was decreased post LPS injection compared with that of the sham controls, however, it was significantly improved in the EGCGtreated LPS-injured group compared with the LPS-injured group with no treatment (Fig. 3A~C). The results indicate that EGCG ameliorated the neuronal differentiation of NSCs impaired by LPS in the adult hippocampal DG at an early stage. In addition, NeuN (a mature neuronal marker) and BrdU-positive cells at 28 days were decreased in the LPS-injured group, whereas the number of double positive cells was increased in the EGCG-treated LPS-injured group compared with the vehicle-treated LPS-injured group (Fig. 4A~C). It is indicating that EGCG had a recovery effect on the neuronal differentiation of adult NSCs at a late stage, which was impaired by LPS induced neuroinflammation. These results demonstrate that the neuronal differentiation of adult NSCs in the DG is rescued by the injection of EGCG into mice with LPS-induced neuroinflammation. Our data also was supported the beneficial effect of EGCG on adult neurogenesis by showing improved proliferation and differentiation of adult mouse hippocampal NSCs in the DG under normal conditions [48].
TLRs are expressed in immune and non-immune cells and are crucial for innate immune responses. For instance, TLR4 is activated by LPS-mediated inflammation in immune cells [49], and is also found in the central nervous system for regulating neurogenesis [50]. A previous report showed that EGCG has anti-inflammatory activity via the downregulation of TLR4 expression [51]. Thus, the recruitment of activated microglia was evaluated in LPS-induced neuroinflammation by showing the number of Iba-1-positive microglial cells. LPS activated the concentrating microglia in the brain, however, the activated microglia were suppressed by EGCG treatment, which modified the TLR4-mediated NF-κB pathway and proinflammatory cytokines (Fig. 5A~G). The results suggest a potential role of EGCG in significantly improving adult neurogenesis impaired by neuroinflammation, and show that the beneficial effect was associated with the downregulation of proinflammatory cytokines through the modulation of microglial activity.
It is still unclear whether EGCG decreases the level of oxidative stress in neuroinflammation for its improvement of adult neurogenesis. Published reports have demonstrated that microglia produce reactive oxygen species (ROS) in PD [5253], and in vivo and in vitro models of AD have shown that EGCG modulates the cellular mechanisms of neuroprotection and neurorestoration through the activation of the protein kinase C pathway for improving cell survival and apoptosis and antioxidant function against ROS [5455]. These works support that EGCG may be involved in the regulation of ROS for rescuing adult neurogenesis affected by neuroinflammation. To effectively address these questions, studies regarding the molecular mechanisms of EGCG in relation to ROS using primary cultures of adult NSCs are required in the future.
In conclusion, we suggest that the administration of EGCG was beneficial for improving the proliferation, survival rate, and neuronal differentiation of adult NSCs in the DG by suppressing the activity of microglia and the TLR4-mediated NF-κB pathway in impaired neurogenesis caused by neuroinflammation. Therefore, EGCG may be a potential therapeutic agent for neuroinflammatory diseases.
ACKNOWLEDGEMENTS
This work was supported by the National Research Foundation of Korea (NRF) grant funded by the Korea government (MSIP) (2011-0030121), (2014R1A2A2A01007582) and by a grant (CRI 12052-22) from Chonnam National University Hospital Biomedical Research Institute.
Notes
Author contributions: K.J.S., H.G.L., J.Y.J. and W.J.K. designed the experiments. Cell cultures, immunohistochemistry and molecular works was performed by S.H.C., H.G.L., H.M.K. and M.S.K. K.J.S. and H.G.L. took care all animals. K.J.S., H.G.L., J.Y.J. and W.J.K. analyzed the data and wrote the manuscript.
References
1. Ekdahl CT, Kokaia Z, Lindvall O. Brain inflammation and adult neurogenesis: the dual role of microglia. Neuroscience. 2009; 158:1021–1029. PMID: 18662748.


2. Polazzi E, Monti B. Microglia and neuroprotection: from in vitro studies to therapeutic applications. Prog Neurobiol. 2010; 92:293–315. PMID: 20609379.


3. Dheen ST, Kaur C, Ling EA. Microglial activation and its implications in the brain diseases. Curr Med Chem. 2007; 14:1189–1197. PMID: 17504139.
4. Lourbopoulos A, Ertürk A, Hellal F. Microglia in action: how aging and injury can change the brain's guardians. Front Cell Neurosci. 2015; 9:54. PMID: 25755635.


5. Casano AM, Peri F. Microglia: multitasking specialists of the brain. Dev Cell. 2015; 32:469–477. PMID: 25710533.


6. Delpech JC, Madore C, Nadjar A, Joffre C, Wohleb ES, Layé S. Microglia in neuronal plasticity: Influence of stress. Neuropharmacology. 2015; 96:19–28. PMID: 25582288.


7. Bilimoria PM, Stevens B. Microglia function during brain development: New insights from animal models. Brain Res. 2015; 1617:7–17. PMID: 25463024.


8. Jeong JW, Lee HH, Han MH, Kim GY, Kim WJ, Choi YH. Anti-inflammatory effects of genistein via suppression of the toll-like receptor 4-mediated signaling pathway in lipopolysaccharide-stimulated BV2 microglia. Chem Biol Interact. 2014; 212:30–39. PMID: 24491678.


9. Su X, Chen Q, Chen W, Chen T, Li W, Li Y, Dou X, Zhang Y, Shen Y, Wu H, Yu C. Mycoepoxydiene inhibits activation of BV2 microglia stimulated by lipopolysaccharide through suppressing NF-κB, ERK 1/2 and toll-like receptor pathways. Int Immunopharmacol. 2014; 19:88–93. PMID: 24447679.


10. Park KW, Lee DY, Joe EH, Kim SU, Jin BK. Neuroprotective role of microglia expressing interleukin-4. J Neurosci Res. 2005; 81:397–402. PMID: 15948189.


11. Zhang Q, Yuan L, Liu D, Wang J, Wang S, Zhang Q, Gong Y, Liu H, Hao A, Wang Z. Hydrogen sulfide attenuates hypoxia-induced neurotoxicity through inhibiting microglial activation. Pharmacol Res. 2014; 84:32–44. PMID: 24788079.


12. Lehnardt S. Innate immunity and neuroinflammation in the CNS: the role of microglia in Toll-like receptor-mediated neuronal injury. Glia. 2010; 58:253–263. PMID: 19705460.


13. Matsuda T, Murao N, Katano Y, Juliandi B, Kohyama J, Akira S, Kawai T, Nakashima K. TLR9 signalling in microglia attenuates seizure-induced aberrant neurogenesis in the adult hippocampus. Nat Commun. 2015; 6:6514. PMID: 25751136.


14. Suhonen JO, Peterson DA, Ray J, Gage FH. Differentiation of adult hippocampus-derived progenitors into olfactory neurons in vivo. Nature. 1996; 383:624–627. PMID: 8857538.


16. Suh H, Deng W, Gage FH. Signaling in adult neurogenesis. Annu Rev Cell Dev Biol. 2009; 25:253–275. PMID: 19575663.


17. Wen S, Li H, Liu J. Dynamic signaling for neural stem cell fate determination. Cell Adh Migr. 2009; 3:107–117. PMID: 19262166.


18. Brown J, Cooper-Kuhn CM, Kempermann G, Van Praag H, Winkler J, Gage FH, Kuhn HG. Enriched environment and physical activity stimulate hippocampal but not olfactory bulb neurogenesis. Eur J Neurosci. 2003; 17:2042–2046. PMID: 12786970.


19. van Praag H, Kempermann G, Gage FH. Running increases cell proliferation and neurogenesis in the adult mouse dentate gyrus. Nat Neurosci. 1999; 2:266–270. PMID: 10195220.


20. Drapeau E, Mayo W, Aurousseau C, Le Moal M, Piazza PV, Abrous DN. Spatial memory performances of aged rats in the water maze predict levels of hippocampal neurogenesis. Proc Natl Acad Sci U S A. 2003; 100:14385–14390. PMID: 14614143.


21. Tatebayashi Y, Lee MH, Li L, Iqbal K, Grundke-Iqbal I. The dentate gyrus neurogenesis: a therapeutic target for Alzheimer's disease. Acta Neuropathol. 2003; 105:225–232. PMID: 12557008.


22. Abrous DN, Koehl M, Le Moal M. Adult neurogenesis: from precursors to network and physiology. Physiol Rev. 2005; 85:523–569. PMID: 15788705.


23. Rogers AE, Hafer LJ, Iskander YS, Yang S. Black tea and mammary gland carcinogenesis by 7,12-dimethylbenz[a]anthracene in rats fed control or high fat diets. Carcinogenesis. 1998; 19:1269–1273. PMID: 9683188.
24. Zhou H, Chen JX, Yang CS, Yang MQ, Deng Y, Wang H. Gene regulation mediated by microRNAs in response to green tea polyphenol EGCG in mouse lung cancer. BMC Genomics. 2014; 15(Suppl 11):S3.


25. Singh BN, Shankar S, Srivastava RK. Green tea catechin, epigallocatechin-3-gallate (EGCG): mechanisms, perspectives and clinical applications. Biochem Pharmacol. 2011; 82:1807–1821. PMID: 21827739.


26. Lecumberri E, Dupertuis YM, Miralbell R, Pichard C. Green tea polyphenol epigallocatechin-3-gallate (EGCG) as adjuvant in cancer therapy. Clin Nutr. 2013; 32:894–903. PMID: 23582951.


27. Steinmann J, Buer J, Pietschmann T, Steinmann E. Anti-infective properties of epigallocatechin-3-gallate (EGCG), a component of green tea. Br J Pharmacol. 2013; 168:1059–1073. PMID: 23072320.


28. Gould E. How widespread is adult neurogenesis in mammals? Nat Rev Neurosci. 2007; 8:481–488. PMID: 17514200.


29. Lledo PM, Alonso M, Grubb MS. Adult neurogenesis and functional plasticity in neuronal circuits. Nat Rev Neurosci. 2006; 7:179–193. PMID: 16495940.


30. Kriegstein A, Alvarez-Buylla A. The glial nature of embryonic and adult neural stem cells. Annu Rev Neurosci. 2009; 32:149–184. PMID: 19555289.


31. Drew LJ, Fusi S, Hen R. Adult neurogenesis in the mammalian hippocampus: why the dentate gyrus? Learn Mem. 2013; 20:710–729. PMID: 24255101.


32. Singhal G, Jaehne EJ, Corrigan F, Toben C, Baune BT. Inflammasomes in neuroinflammation and changes in brain function: a focused review. Front Neurosci. 2014; 8:315. PMID: 25339862.


33. Monje ML, Toda H, Palmer TD. Inflammatory blockade restores adult hippocampal neurogenesis. Science. 2003; 302:1760–1765. PMID: 14615545.


34. Filiou MD, Arefin AS, Moscato P, Graeber MB. 'Neuroinflammation' differs categorically from inflammation: transcriptomes of Alzheimer's disease, Parkinson's disease, schizophrenia and inflammatory diseases compared. Neurogenetics. 2014; 15:201–212. PMID: 24928144.


35. Song C, Wang H. Cytokines mediated inf lammation and decreased neurogenesis in animal models of depression. Prog Neuropsychopharmacol Biol Psychiatry. 2011; 35:760–768. PMID: 20600462.
36. Roy AM, Baliga MS, Katiyar SK. Epigallocatechin-3-gallate induces apoptosis in estrogen receptor-negative human breast carcinoma cells via modulation in protein expression of p53 and Bax and caspase-3 activation. Mol Cancer Ther. 2005; 4:81–90. PMID: 15657356.
37. Abd El Mohsen MM, Kuhnle G, Rechner AR, Schroeter H, Rose S, Jenner P, Rice-Evans CA. Uptake and metabolism of epicatechin and its access to the brain after oral ingestion. Free Radic Biol Med. 2002; 33:1693–1702. PMID: 12488137.


38. Lin LC, Wang MN, Tseng TY, Sung JS, Tsai TH. Pharmacokinetics of (-)-epigallocatechin-3-gallate in conscious and freely moving rats and its brain regional distribution. J Agric Food Chem. 2007; 55:1517–1524. PMID: 17256961.


39. Li J, Ye L, Wang X, Liu J, Wang Y, Zhou Y, Ho W. (-)-Epigallocatechin gallate inhibits endotoxin-induced expression of inflammatory cytokines in human cerebral microvascular endothelial cells. J Neuroinflammation. 2012; 9:161. PMID: 22768975.


40. Mandel SA, Amit T, Weinreb O, Reznichenko L, Youdim MB. Simultaneous manipulation of multiple brain targets by green tea catechins: a potential neuroprotective strategy for Alzheimer and Parkinson diseases. CNS Neurosci Ther. 2008; 14:352–365. PMID: 19040558.


41. Avramovich-Tirosh Y, Reznichenko L, Mit T, Zheng H, Fridkin M, Weinreb O, Mandel S, Youdim MB. Neurorescue activity, APP regulation and amyloid-beta peptide reduction by novel multifunctional brain permeable iron-chelating-antioxidants, M-30 and green tea polyphenol, EGCG. Curr Alzheimer Res. 2007; 4:403–411. PMID: 17908043.
42. Herges K, Millward JM, Hentschel N, Infante-Duarte C, Aktas O, Zipp F. Neuroprotective effect of combination therapy of glatiramer acetate and epigallocatechin-3-gallate in neuroinflammation. PLoS One. 2011; 6:e25456. PMID: 22022398.


43. Wu KJ, Hsieh MT, Wu CR, Wood WG, Chen YF. Green tea extract ameliorates learning and memory deficits in ischemic rats via its active component polyphenol epigallocatechin-3-gallate by modulation of oxidative stress and neuroinflammation. Evid Based Complement Alternat Med. 2012; 2012:163106. PMID: 22919410.


44. Weinreb O, Amit T, Youdim MB. A novel approach of proteomics and transcriptomics to study the mechanism of action of the antioxidant-iron chelator green tea polyphenol (-)-epigallocatechin-3-gallate. Free Radic Biol Med. 2007; 43:546–556. PMID: 17640565.


45. Weinreb O, Mandel S, Amit T, Youdim MB. Neurological mechanisms of green tea polyphenols in Alzheimer's and Parkinson's diseases. J Nutr Biochem. 2004; 15:506–516. PMID: 15350981.


46. Gan L, Meng ZJ, Xiong RB, Guo JQ, Lu XC, Zheng ZW, Deng YP, Luo BD, Zou F, Li H. Green tea polyphenol epigallocatechin-3-gallate ameliorates insulin resistance in non-alcoholic fatty liver disease mice. Acta Pharmacol Sin. 2015; 36:597–605. PMID: 25891086.


47. Wang Y, Li M, Xu X, Song M, Tao H, Bai Y. Green tea epigallocatechin-3-gallate (EGCG) promotes neural progenitor cell proliferation and sonic hedgehog pathway activation during adult hippocampal neurogenesis. Mol Nutr Food Res. 2012; 56:1292–1303. PMID: 22692966.


48. Yoo KY, Choi JH, Hwang IK, Lee CH, Lee SO, Han SM, Shin HC, Kang IJ, Won MH. (-)-Epigallocatechin-3-gallate increases cell proliferation and neuroblasts in the subgranular zone of the dentate gyrus in adult mice. Phytother Res. 2010; 24:1065–1070. PMID: 20013823.


49. Wang W, Deng M, Liu X, Ai W, Tang Q, Hu J. TLR4 activation induces nontolerant inf lammatory response in endothelial cells. Inflammation. 2011; 34:509–518. PMID: 20878353.
50. Rolls A, Shechter R, London A, Ziv Y, Ronen A, Levy R, Schwartz M. Toll-like receptors modulate adult hippocampal neurogenesis. Nat Cell Biol. 2007; 9:1081–1088. PMID: 17704767.


51. Hong Byun E, Fujimura Y, Yamada K, Tachibana H. TLR4 signaling inhibitory pathway induced by green tea polyphenol epigallocatechin-3-gallate through 67-kDa laminin receptor. J Immunol. 2010; 185:33–45. PMID: 20511545.


52. Kumar P, Kumar A. Protective effects of epigallocatechin gallate following 3-nitropropionic acid-induced brain damage: possible nitric oxide mechanisms. Psychopharmacology (Berl). 2009; 207:257–270. PMID: 19763544.


53. Mao H, Fang X, Floyd KM, Polcz JE, Zhang P, Liu B. Induction of microglial reactive oxygen species production by the organochlorinated pesticide dieldrin. Brain Res. 2007; 1186:267–274. PMID: 17999924.


54. Levites Y, Amit T, Youdim MB, Mandel S. Involvement of protein kinase C activation and cell survival/cell cycle genes in green tea polyphenol (-)-epigallocatechin 3-gallate neuroprotective action. J Biol Chem. 2002; 277:30574–30580. PMID: 12058035.
55. Weinreb O, Amit T, Mandel S, Youdim MB. Neuroprotective molecular mechanisms of (-)-epigallocatechin-3-gallate: a reflective outcome of its antioxidant, iron chelating and neuritogenic properties. Genes Nutr. 2009; 4:283–296. PMID: 19756809.


SUPPLEMENTARY MATERIALS
Supplementary data including one figure can be found with this article online at http://pdf.medrang.co.kr/paper/pdf/Kjpp/Kjpp020-01-06-s001.pdf.
Supplementary figure 1
The schematic representation of experimental research design.
(A) LPS (black arrow), EGCG (red arrows, 3 times with 6 hours interval), and BrdU (gray arrow) injection were done in the time course and the animals were sacrificed on day 1, 3, and 5 for immunohistochemical analysis to the quantification of NSC proliferation and immature differentiation. (B) LPS (black arrow), EGCG (red arrows), and 5 consecutive BrdU (gray arrows) injection daily were done in the time course and the animals were sacrificed on day 5 and 28 for immunohistochemical analysis to the quantification of NSC cell survival and mature differentiation. LPS, lipopolysaccharide, EGCG, epigallocatechin-3-gallate, BrdU, bromodeoxyuridine, injt, injection, and Sac, scarified.
Fig. 1
The effect of EGCG on the proliferation of adult NSCs in the DG impaired by LPS-induced neuroinflammation.
(A) Representative images show BrdU-positive cells in the adult dentate gyrus (DG) on day 1 and day 3 after BrdU injection following LPS injection. Newly proliferated cells in the subgranular zone (SGZ) of the DG were immunolabeled using anti-BrdU (green). (B, C) Quantitative analysis of the number of BrdU-positive cells in the DG of the hippocampus on day 1 and day 3 after LPS injection. (n=5 each). BrdU-positive cells were decreased in the LPS-injured group at 1st, and 3rd day post brain inflammation, which was improved significantly by EGCG (p=0.0357 and p=0.0404 respectively, one-way ANOVA) (*p<0.05, ***p<0.001). Data are expressed as the mean±SEM. Scale bar, 200 µm.
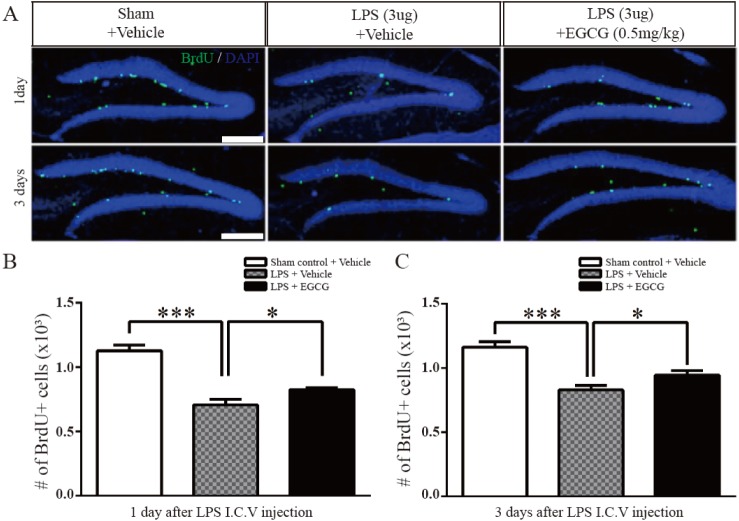
Fig. 2
The effect of EGCG on the immature neuronal differentiation in the DG after LPS-induced neuroinflammation.
(A) Representative images show BrdU- and DCX-positive cells in the DG 5 days after BrdU injection following LPS injection. BrdU (green) and DCX (red) double stained cells (yellow) represent newly generated immature neurons (arrows). (B, C) Quantitative analysis of the number of BrdU-positive cells and/or DCX-positive cells (n=5 each). The differentiation of the NSCs in the hippocampal DG affected by LPS-induced neuroinflammation was recovered by EGCG (0.5 mg/kg) (p=0.0302, one-way ANOVA) (*p<0.05, **p<0.01). Data are expressed as the mean±SEM. Scale bar, 200 µm.
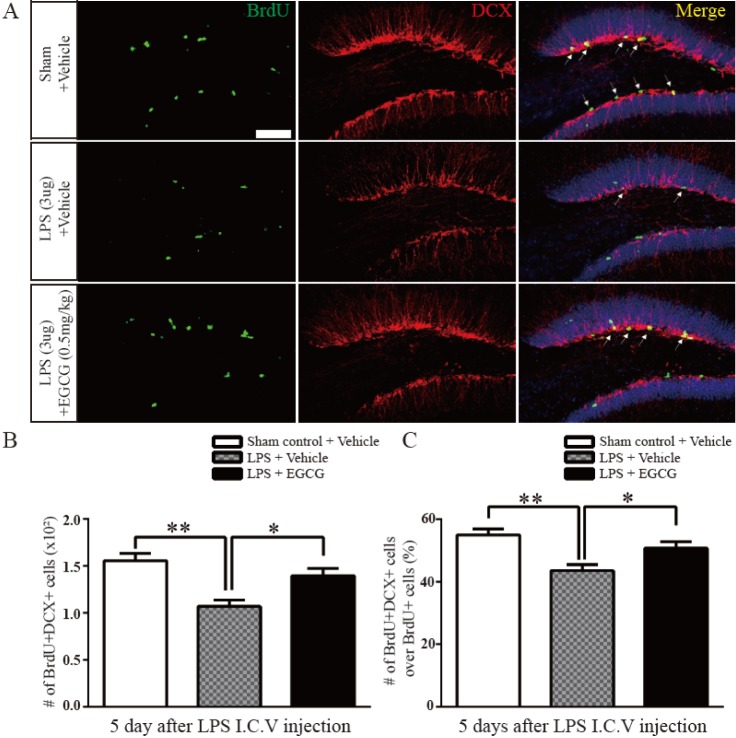
Fig. 3
The effect of EGCG on the mature neuronal differentiation in the DG after LPS-induced neuroinflammation.
(A) Representative images show BrdU and NeuN double immunostained cells in the DG 28 days after BrdU injection following LPS injection. BrdU (green) and NeuN (red) double immunostained cells (yellow) represent newly generated mature neurons (arrows). (B, C) Quantitative analysis of the number of BrdU- and/or NeuN-positive cells (n=5 each). BrdU and NeuN double positive cells represent matured neurons from adult NSCs that are affected by neuroinflammation, and the damage was rescued by EGCG (0.5 mg/kg) (p=0.0378, one-way ANOVA) (*p<0.05, **p<0.01). Data are expressed as the mean±SEM. Scale bar, 200 µm.
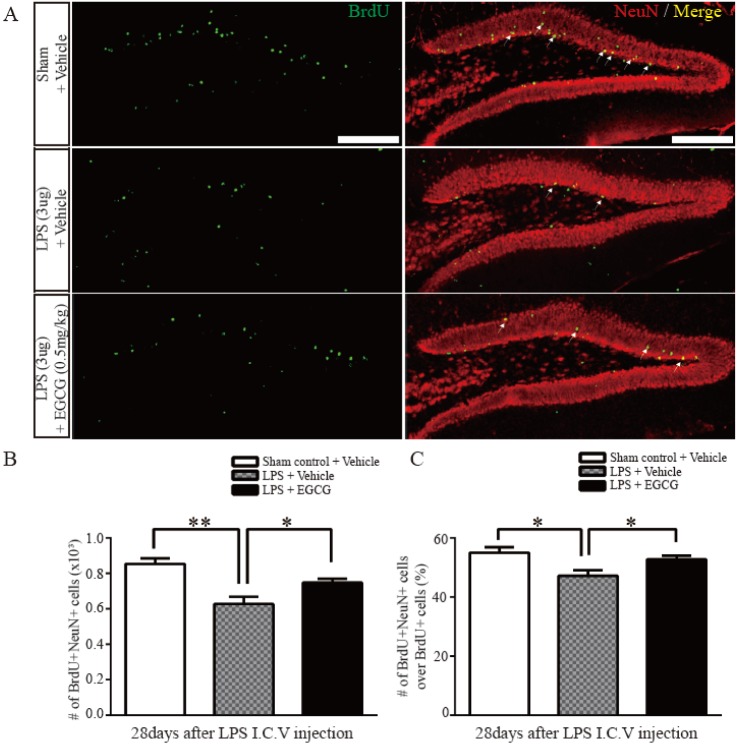
Fig. 4
The effect of EGCG on the survival of newborn cells in the DG after LPS-induced neuroinflammation.
(A) Representative images show BrdU-positive cells (green) in the DG at 3 hours (upper) or 28 days (lower) after consecutive BrdU injection for 5 days, following LPS injection. (B) Representative images show cleaved caspase-3 immunoreactive cells (red) in the DG 28 days after consecutive BrdU injection for 5 days. (C) The survival rate of newborn cells in LPS-induced injures was improved significantly by EGCG (0.5 mg/kg) which was analyzed by counting BrdU-positive cells in the DG of the hippocampus at 5 days over 28 days (n =5 each, p=0.0170, one-way ANOVA) (*p<0.05, **p<0.01). (D) Quantitative analysis of the number of cleaved caspase-3-positive cells in the DG of the hippocampus (n=5 each). The number of cleaved caspase-3-positive cells in the DG of the hippocampus was decreased in the EGCG-treated LPS-injured group (p=0.0109, one-way ANOVA) (*p<0.05). Data are expressed as the mean±SEM; Scale bar in A and B, 200 µm.
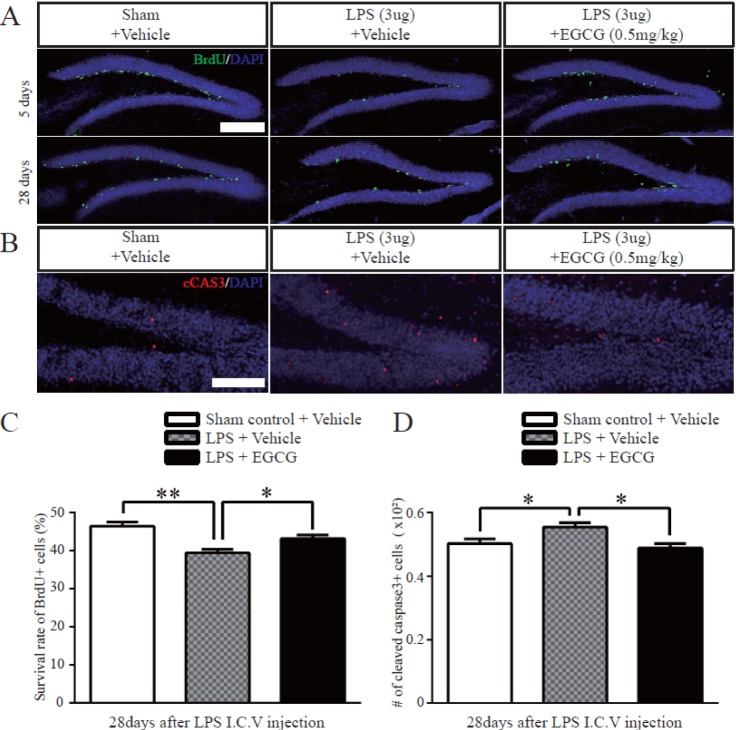
Fig. 5
The effect of EGCG on microglia and proinflammatory cytokines after LPS-induced neuroinflammation.
(A) Representative images show Iba-1-positive cells (green) in the DG on day 1 (upper) and day 3 (lower) after LPS injection. (B) The positive effect of EGCG was quantified by counting the number of Iba-1-positive cells that was significantly reduced by EGCG (0.5 mg/kg) compared with LPS only (n=5 each, day 1; p=0.0002, day 3; p=0.0025 respectively, one-way ANOVA). (C) Western blotting analysis was performed to quantify the TLR4, Rel A, active form of Rel A (pRel A), and β-actin as a control in the hippocampal DG. The TLR4-NFκB pathway was increased by LPS, which was rescued by EGCG (0.5 mg/kg) (n=4 each). (D~G) Quantitative real-time PCR and ELISA was performed to measure IL-1β, IL-6, and TNF-α in the hippocampus after LPS-induced neuroinflammation (n=4 each). The levels of IL-1β, IL-6, and TNF-α were suppressed by EGCG treatment in LPS-induced (n=4 each) p<0.05, **p<0.01 compared with the control, ⋆p<0.05 and ⋆⋆p< 0.01 compared with the LPS-injured group, ***p<0.001). Data are expressed as the mean±SEM. Scale bar in A, 200 µm (upper) and in an inset, 100 µm (lower).
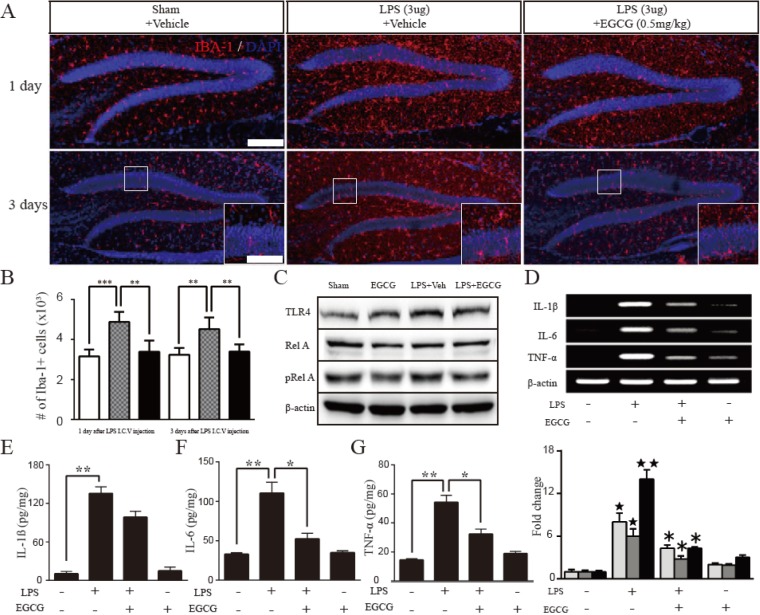