Abstract
We carried out a series of experiment demonstrating the role of mitochondria in the cytosolic and mitochondrial Ca2+ transients and compared the results with those from computer simulation. In rat ventricular myocytes, increasing the rate of stimulation (1∼3 Hz) made both the diastolic and systolic [Ca2+] bigger in mitochondria as well as in cytosol. As L-type Ca2+ channel has key influence on the amplitude of Ca2+-induced Ca2+ release, the relation between stimulus frequency and the amplitude of Ca2+ transients was examined under the low density (1/10 of control) of L-type Ca2+ channel in model simulation, where the relation was reversed. In experiment, block of Ca2+ uniporter on mitochondrial inner membrane significantly reduced the amplitude of mitochondrial Ca2+ transients, while it failed to affect the cytosolic Ca2+ transients. In computer simulation, the amplitude of cytosolic Ca2+ transients was not affected by removal of Ca2+ uniporter. The application of carbonyl cyanide 4-(trifluoromethoxy) phenylhydrazone (FCCP) known as a protonophore on mitochondrial membrane to rat ventricular myocytes gradually increased the diastolic [Ca2+] in cytosol and eventually abolished the Ca2+ transients, which was similarly reproduced in computer simulation. The model study suggests that the relative contribution of L-type Ca2+ channel to total transsarcolemmal Ca2+ flux could determine whether the cytosolic Ca2+ transients become bigger or smaller with higher stimulus frequency. The present study also suggests that cytosolic Ca2+ affects mitochondrial Ca2+ in a beat-to-beat manner, however, removal of Ca2+ influx mechanism into mitochondria does not affect the amplitude of cytosolic Ca2+ transients.
References
1. Sakamoto J, Tonomura Y. Order of release of ADP and Pi from phosphoenzyme with bound ADP of Ca2+-dependent ATPase from sarcoplasmic reticulum and of Na+, K+-dependent ATPase studied by ADP-inhibition patterns. J Biochem. 1980; 87:1721–1727.
2. Jensen AM, S⊘rensen TL, Olesen C, M⊘ller JV, Nissen P. Modulatory and catalytic modes of ATP binding by the calcium pump. EMBO J. 2006; 25:2305–2314.


3. Wan B, Doumen C, Duszynski J, Salama G, Vary TC, LaNoue KF. Effects of cardiac work on electrical potential gradient across mitochondrial membrane in perfused rat hearts. Am J Physiol. 1993; 265:H453–460.


4. Territo PR, Mootha VK, French SA, Balaban RS. Ca2+ activation of heart mitochondrial oxidative phosphorylation: role of the F0/F1-ATPase. Am J Physiol Cell Physiol. 2000; 278:C423–435.
5. McCormack JG, Halestrap AP, Denton RM. Role of calcium ions in regulation of mammalian intramitochondrial metabolism. Physiol Rev. 1990; 70:391–425.


6. Hansford RG. Dehydrogenase activation by Ca2+ in cells and tissues. J Bioenerg Biomembr. 1991; 23:823–854.
7. Brandes R, Bers DM. Simultaneous measurements of mitochondrial NADH and Ca2+ during increased work in intact rat heart trabeculae. Biophys J. 2002; 83:587–604.
8. Trollinger DR, Cascio WE, Lemasters JJ. Mitochondrial calcium transients in adult rabbit cardiac myocytes: inhibition by ruthenium red and artifacts caused by lysosomal loading of Ca2+-indicating fluorophores. Biophys J. 2000; 79:39–50.
9. Andrienko TN, Picht E, Bers DM. Mitochondrial free calcium regulation during sarcoplasmic reticulum calcium release in rat cardiac myocytes. J Mol Cell Cardiol. 2009; 46:1027–1036.


10. Sedova M, Dedkova EN, Blatter LA. Integration of rapid cytosolic Ca2+ signals by mitochondria in cat ventricular myocytes. Am J Physiol Cell Physiol. 2006; 291:C840–850.
11. Matsuoka S, Sarai N, Kuratomi S, Ono K, Noma A. Role of individual ionic current systems in ventricular cells hypothesized by a model study. Jpn J Physiol. 2003; 53:105–123.


12. Cortassa S, Aon MA, O'Rourke B, Jacques R, Tseng HJ, Marbán E, Winslow RL. A computational model integrating electrophysiology, contraction, and mitochondrial bioenergetics in the ventricular myocyte. Biophys J. 2006; 91:1564–1589.


13. Bernardi P. Mitochondrial transport of cations: channels, exchangers, and permeability transition. Physiol Rev. 1999; 79:1127–1155.


14. Pandit SV, Clark RB, Giles WR, Demir SS. A mathematical model of action potential heterogeneity in adult rat left ventricular myocytes. Biophys J. 2001; 81:3029–3051.


15. Kang SH, Park WS, Kim N, Youm JB, Warda M, Ko JH, Ko EA, Han J. Mitochondrial Ca2+-activated K+ channels more efficiently reduce mitochondrial Ca2+ overload in rat ventricular myocytes. Am J Physiol Heart Circ Physiol. 2007; 293:H307–313.
16. Youm JB, Jo SH, Leem CH, Ho WK, Earm YE. Role of stretch-activated channels in stretch-induced changes of electrical activity in rat atrial myocytes. Korean J Physiol Pharmacol. 2004; 8:33–41.
17. Katsube Y, Yokoshiki H, Nguyen L, Yamamoto M, Sperelakis N. L-type Ca2+ currents in ventricular myocytes from neonatal and adult rats. Can J Physiol Pharmacol. 1998; 76:873–881.


18. Berlin JR, Bassani JW, Bers DM. Intrinsic cytosolic calcium buffering properties of single rat cardiac myocytes. Biophys J. 1994; 67:1775–1787.


19. Page E. Quantitative ultrastructural analysis in cardiac membrane physiology. Am J Physiol. 1978; 235:C147–158.


20. Shimoni Y, Severson D, Giles W. Thyroid status and diabetes modulate regional differences in potassium currents in rat ventricle. J Physiol. 1995; 488:673–688.


21. Nie A, Meng Z. Sulfur dioxide derivative modulation of potassium channels in rat ventricular myocytes. Arch Biochem Biophys. 2005; 442:187–195.


22. Apkon M, Nerbonne JM. Characterization of two distinct depolarization-activated K+ currents in isolated adult rat ventricular myocytes. J Gen Physiol. 1991; 97:973–1011.
23. Wettwer E, Amos G, Gath J, Zerkowski HR, Reidemeister JC, Ravens U. Transient outward current in human and rat ventricular myocytes. Cardiovasc Res. 1993; 27:1662–1669.


24. Volk T, Nguyen TH, Schultz JH, Faulhaber J, Ehmke H. Regional alterations of repolarizing K+ currents among the left ventricular free wall of rats with ascending aortic stenosis. J Physiol. 2001; 530:443–455.
25. Pond AL, Scheve BK, Benedict AT, Petrecca K, Van Wagoner DR, Shrier A, Nerbonne JM. Expression of distinct ERG proteins in rat, mouse, and human heart. Relation to functional IKr channels. J Biol Chem. 2000; 275:5997–6006.
26. Sakmann B, Trube G. Conductance properties of single inwardly rectifying potassium channels in ventricular cells from guinea-pig heart. J Physiol. 1984; 347:641–657.


27. Fauconnier J, Lacampagne A, Rauzier JM, Vassort G, Richard S. Ca2+-dependent reduction of IK1 in rat ventricular cells: a novel paradigm for arrhythmia in heart failure? Cardiovasc Res. 2005; 68:204–212.
28. Nichols CG, Lederer WJ. The regulation of ATP-sensitive K+ channel activity in intact and permeabilized rat ventricular myocytes. J Physiol. 1990; 423:91–110.
29. Luo CH, Rudy Y. A dynamic model of the cardiac ventricular action potential. I. Simulations of ionic currents and concentration changes. Circ Res. 1994; 74:1071–1096.


30. Brown AM, Lee KS, Powell T. Sodium current in single rat heart muscle cells. J Physiol. 1981; 318:479–500.


31. Bogdanov KY, Ziman BD, Spurgeon HA, Lakatta EG. L- and T-type calcium currents differ in finch and rat ventricular cardiomyocytes. J Mol Cell Cardiol. 1995; 27:2581–2593.
32. Zühlke RD, Pitt GS, Deisseroth K, Tsien RW, Reuter H. Calmodulin supports both inactivation and facilitation of L-type calcium channels. Nature. 1999; 399:159–162.


33. Sun L, Fan JS, Clark JW, Palade PT. A model of the L-type Ca2+ channel in rat ventricular myocytes: ion selectivity and inactivation mechanisms. J Physiol. 2000; 529:139–158.
34. Youm JB, Han J, Kim N, Zhang YH, Kim E, Joo H, Hun Leem C, Joon Kim S, Cha KA, Earm YE. Role of stretch-activated channels on the stretch-induced changes of rat atrial myocytes. Prog Biophys Mol Biol. 2006; 90:186–206.


35. Coulombe A, Lefèvre IA, Baro I, Coraboeuf E. Barium- and calcium-permeable channels open at negative membrane potentials in rat ventricular myocytes. J Membr Biol. 1989; 111:57–67.


36. Wang SY, Clague JR, Langer GA. Increase in calcium leak channel activity by metabolic inhibition or hydrogen peroxide in rat ventricular myocytes and its inhibition by polycation. J Mol Cell Cardiol. 1995; 27:211–222.


37. Bers DM. Ca influx and sarcoplasmic reticulum Ca release in cardiac muscle activation during postrest recovery. Am J Physiol. 1985; 248:H366–381.


38. Dibb KM, Eisner DA, Trafford AW. Regulation of systolic [Ca2+]i and cellular Ca2+ flux balance in rat ventricular myocytes by SR Ca2+, L-type Ca2+ current and diastolic [Ca2+]i. J Physiol. 2007; 585:579–592.
39. Harrison SM, McCall E, Boyett MR. The relationship between contraction and intracellular sodium in rat and guinea-pig ventricular myocytes. J Physiol. 1992; 449:517–550.


40. Niederer SA, Smith NP. A mathematical model of the slow force response to stretch in rat ventricular myocytes. Biophys J. 2007; 92:4030–4044.


41. Negroni JA, Lascano EC. A cardiac muscle model relating sarcomere dynamics to calcium kinetics. J Mol Cell Cardiol. 1996; 28:915–929.


42. Magnus G, Keizer J. Minimal model of beta-cell mitochondrial Ca2+ handling. Am J Physiol. 1997; 273:C717–733.
43. Nguyen MH, Dudycha SJ, Jafri MS. Effect of Ca2+ on cardiac mitochondrial energy production is modulated by Na+ and H+ dynamics. Am J Physiol Cell Physiol. 2007; 292:C2004–2020.
44. Jung DW, Apel LM, Brierley GP. Transmembrane gradients of free Na+ in isolated heart mitochondria estimated using a fluorescent probe. Am J Physiol. 1992; 262:C1047–1055.
45. de la Fuente S, Montenegro P, Fonteriz RI, Moreno A, Lobatón CD, Montero M, Alvarez J. The dynamics of mitochondrial Ca2+ fluxes. Biochim Biophys Acta. 2010; 1797:1727–1735.
46. Linz KW, Meyer R. Control of L-type calcium current during the action potential of guinea-pig ventricular myocytes. J Physiol. 1998; 513:425–442.


47. Koch-Weser J, Blinks JR. The influence of the interval between beats on myocardial contractility. Pharmacol Rev. 1963; 15:601–652.
48. Capogrossi MC, Kort AA, Spurgeon HA, Lakatta EG. Single adult rabbit and rat cardiac myocytes retain the Ca2+- and species-dependent systolic and diastolic contractile properties of intact muscle. J Gen Physiol. 1986; 88:589–613.
49. Borzak S, Murphy S, Marsh JD. Mechanisms of rate staircase in rat ventricular cells. Am J Physiol. 1991; 260:H884–892.


50. Frampton JE, Orchard CH, Boyett MR. Diastolic, systolic and sarcoplasmic reticulum [Ca2+] during inotropic interventions in isolated rat myocytes. J Physiol. 1991; 437:351–375.
51. Clark RB, Bouchard RA, Salinas-Stefanon E, Sanchez-Chapula J, Giles WR. Heterogeneity of action potential waveforms and potassium currents in rat ventricle. Cardiovasc Res. 1993; 27:1795–1799.


52. Reed KC, Bygrave FL. The inhibition of mitochondrial calcium transport by lanthanides and ruthenium red. Biochem J. 1974; 140:143–155.


53. Gunter TE, Pfeiffer DR. Mechanisms by which mitochondria transport calcium. Am J Physiol. 1990; 258:C755–786.


54. Sparagna GC, Gunter KK, Sheu SS, Gunter TE. Mitochondrial calcium uptake from physiological-type pulses of calcium. A description of the rapid uptake mode. J Biol Chem. 1995; 270:27510–27515.
55. Beutner G, Sharma VK, Lin L, Ryu SY, Dirksen RT, Sheu SS. Type 1 ryanodine receptor in cardiac mitochondria: transducer of excitation-metabolism coupling. Biochim Biophys Acta. 2005; 1717:1–10.


56. Li W, Shariat-Madar Z, Powers M, Sun X, Lane RD, Garlid KD. Reconstitution, identification, purification, and immunological characterization of the 110-kDa Na+/Ca2+ antiporter from beef heart mitochondria. J Biol Chem. 1992; 267:17983–17989.
57. Vay L, Hernández-SanMiguel E, Lobatón CD, Moreno A, Montero M, Alvarez J. Mitochondrial free [Ca2+] levels and the permeability transition. Cell Calcium. 2009; 45:243–250.
58. Hoffman BF, Kelly JJ Jr. Effects of rate and rhythm on contraction of rat papillary muscle. Am J Physiol. 1959; 197:1199–1204.


59. Kort AA, Lakatta EG. Spontaneous sarcoplasmic reticulum calcium release in rat and rabbit cardiac muscle: relation to transient and rested-state twitch tension. Circ Res. 1988; 63:969–979.


60. Shim EB, Leem CH, Abe Y, Noma A. A new multi-scale simulation model of the circulation: from cells to system. Philos Transact A Math Phys Eng Sci. 2006; 364:1483–1500.
61. Maack C, Cortassa S, Aon MA, Ganesan AN, Liu T, O'Rourke B. Elevated cytosolic Na+ decreases mitochondrial Ca2+ uptake during excitation-contraction coupling and impairs energetic adaptation in cardiac myocytes. Circ Res. 2006; 99:172–182.
62. Robert V, Gurlini P, Tosello V, Nagai T, Miyawaki A, Di Lisa F, Pozzan T. Beat-to-beat oscillations of mitochondrial [Ca2+] in cardiac cells. EMBO J. 2001; 20:4998–5007.
63. Griffiths EJ, Wei SK, Haigney MC, Ocampo CJ, Stern MD, Silverman HS. Inhibition of mitochondrial calcium efflux by clonazepam in intact single rat cardiomyocytes and effects on NADH production. Cell Calcium. 1997; 21:321–329.


64. Bassani RA, Bassani JW, Bers DM. Mitochondrial and sarcolemmal Ca2+ transport reduce [Ca2+]i during caffeine contractures in rabbit cardiac myocytes. J Physiol. 1992; 453:591–608.
65. Bassani JW, Bassani RA, Bers DM. Relaxation in rabbit and rat cardiac cells: species-dependent differences in cellular mechanisms. J Physiol. 1994; 476:279–293.


66. Negretti N, O'Neill SC, Eisner DA. The relative contributions of different intracellular and sarcolemmal systems to relaxation in rat ventricular myocytes. Cardiovasc Res. 1993; 27:1826–1830.


67. Bassani RA, Bassani JW, Bers DM. Relaxation in ferret ventricular myocytes: unusual interplay among calcium transport systems. J Physiol. 1994; 476:295–308.


68. Pacher P, Csordás P, Schneider T, Hajnóczky G. Quantification of calcium signal transmission from sarco-endoplasmic reticulum to the mitochondria. J Physiol. 2000; 529:553–564.


69. Heytler PG. Uncouplers of oxidative phosphorylation. Methods Enzymol. 1979; 55:462–472.
70. Saotome M, Katoh H, Satoh H, Nagasaka S, Yoshihara S, Terada H, Hayashi H. Mitochondrial membrane potential modulates regulation of mitochondrial Ca2+ in rat ventricular myocytes. Am J Physiol Heart Circ Physiol. 2005; 288:H1820–1828.
71. Goldhaber JI, Parker JM, Weiss JN. Mechanisms of excitation-contraction coupling failure during metabolic inhibition in guinea-pig ventricular myocytes. J Physiol. 1991; 443:371–386.


72. Gunter TE, Gunter KK, Sheu SS, Gavin CE. Mitochondrial calcium transport: physiological and pathological relevance. Am J Physiol. 1994; 267:C313–339.


73. Yuan XJ, Sugiyama T, Goldman WF, Rubin LJ, Blaustein MP. A mitochondrial uncoupler increases KCa currents but decreases KV currents in pulmonary artery myocytes. Am J Physiol. 1996; 270:C321–331.
74. Zablockaite D, Gendviliene V, Martisiene I, Jurevicius J. Effect of oxidative phosphorylation uncoupler FCCP and F1F0-ATPase inhibitor oligomycin on the electromechanical activity of human myocardium. Adv Med Sci. 2007; 52:89–93.
75. Higgins TJ, Bailey PJ. The effects of cyanide and iodoacetate intoxication and ischaemia on enzyme release from the perfused rat heart. Biochim Biophys Acta. 1983; 762:67–75.


76. Weiss J, Hiltbrand B. Functional compartmentation of glycolytic versus oxidative metabolism in isolated rabbit heart. J Clin Invest. 1985; 75:436–447.


77. Weiss JN, Lamp ST. Glycolysis preferentially inhibits ATP-sensitive K+ channels in isolated guinea pig cardiac myocytes. Science. 1987; 238:67–69.
78. Jeremy RW, Koretsune Y, Marban E, Becker LC. Relation between glycolysis and calcium homeostasis in postischemic myocardium. Circ Res. 1992; 70:1180–1190.


79. Stanley WC, Recchia FA, Lopaschuk GD. Myocardial substrate metabolism in the normal and failing heart. Physiol Rev. 2005; 85:1093–1129.


82. Brennan JP, Southworth R, Medina RA, Davidson SM, Duchen MR, Shattock MJ. Mitochondrial uncoupling, with low concentration FCCP, induces ROS-dependent cardioprotection independent of KATP channel activation. Cardiovasc Res. 2006; 72:313–321.
Fig. 1.
Comparison of APs generated by experiment (A) and model simulation (B). (A) Myocytes were stimulated at a rate of 1 Hz and a representative trace of AP was obtained after 200 sec of stabilization period. Resting membrane potential was at –76.3±3.7 mV (n=21). APD50 and APD90 of the representative recording were 21.1 ms and 42.9 ms, respectively. Recordings were obtained at room temperature (22∼25°C). (B) Model-generated AP. Resting membrane potential was at –80.2 mV. APD50 and APD90 were 22.2 ms and 43.4 ms, respectively. Temperature was set at 24°C (Table 1).
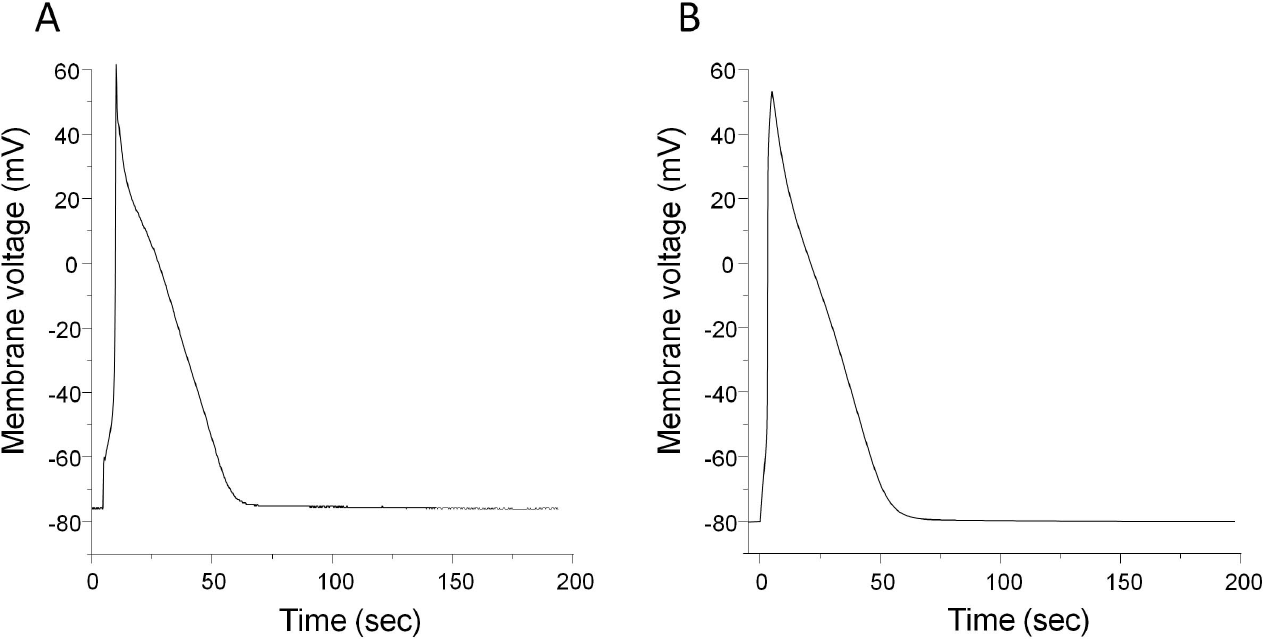
Fig. 2.
Stimulus frequency-dependent change in cytosolic Ca2+ transients recorded by experiment (A) and model (B). (A) Myocytes were field stimulated at a rate of 0.2 Hz for 200 sec and the rate was sequentially changed from 0.2 to 1 Hz, 2 Hz, and 3 Hz. Fura-2 fluorescence ratio (F340/F380) was recorded to see change in cytosolic Ca2+ transients. Expanded trace of fluorescence ratio during 2 Hz stimulation was demonstrated on the right panel. The expanded trace was chosen from our other experimental data. (B) Model-generated cytosolic Ca2+ transients. Expanded trace during 2 Hz stimulation was also displayed on the right panel. The downward arrow indicates the point where the expanded trace was extracted.
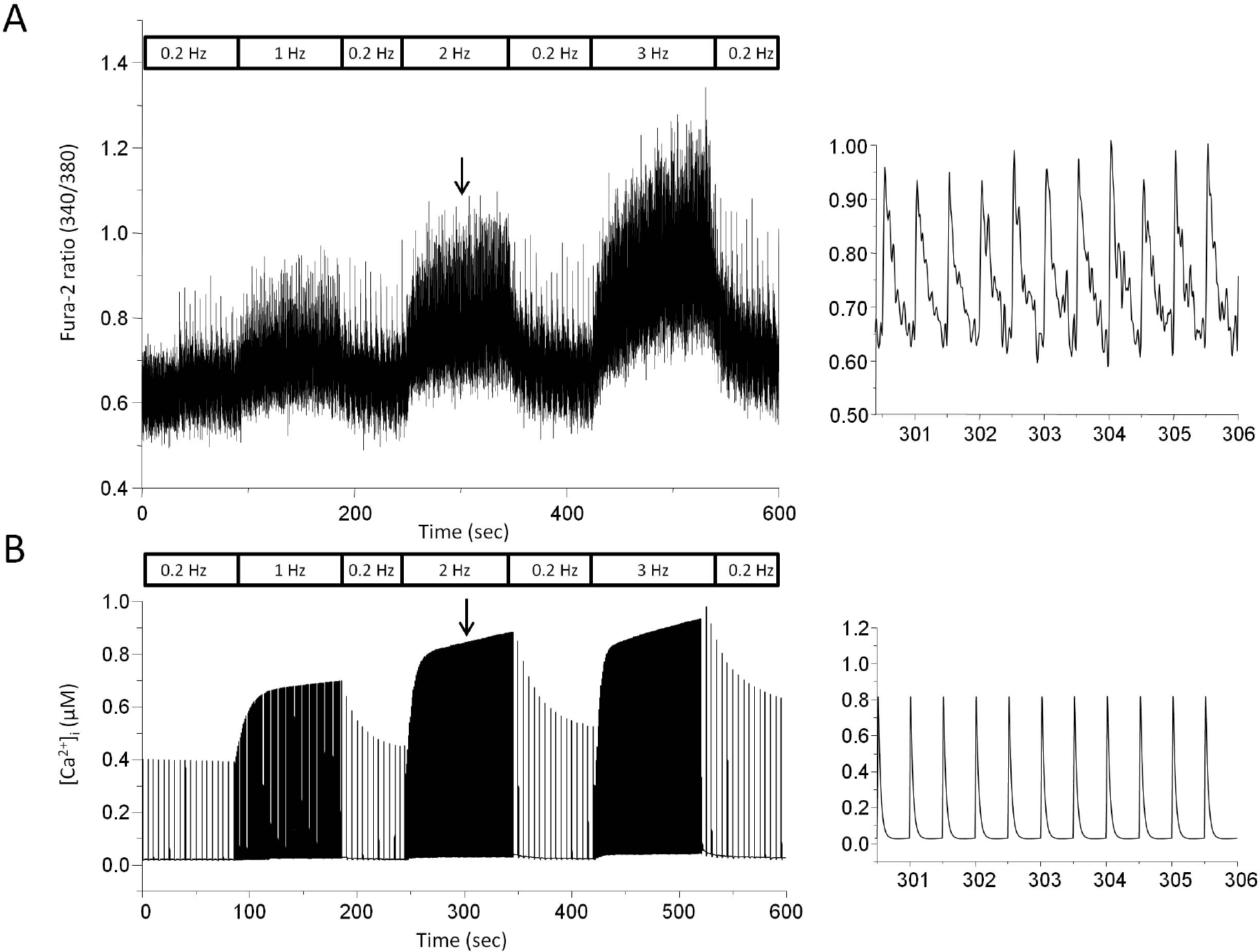
Fig. 3.
Negative staircase in the model with reduced ICaL. (A) Model simulation with control. The rate of stimulation was switched between 0.2 and 0.8 Hz. (B) Model simulation with reduced ICaL. Channel density of L-type Ca2+ channel was reduced to 1/10 of control. The stimulation protocol is the same as that one used in A. (C) Model simulation with increased Ito. Channel density of Ca2+-independent transient outward K+ current (Ito) was increased to 10-times of control.
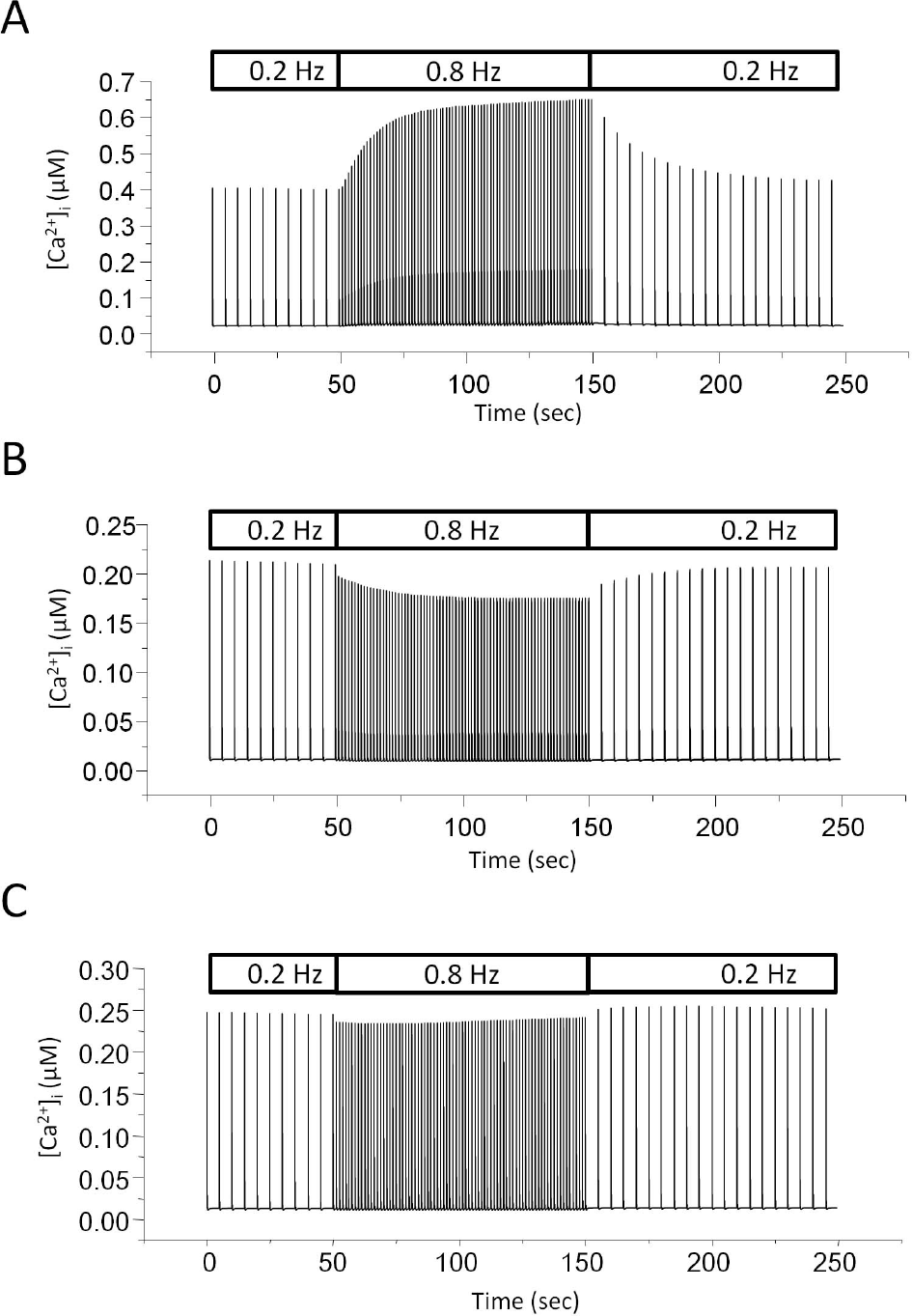
Fig. 4.
Stimulus frequency-dependent change in mitochondrial Ca2+ transients by experiment (A) and model simulation (B). (A) Myocytes were field stimulated and the rate was switched between 0.2 Hz and test frequency (1 Hz, 2 Hz, and 3 Hz). Rhod-2 intensity was recorded to monitor change in mitochondrial Ca2+ transients. Output intensity was normalized to diastolic level at control in order to get a pseudo ratio unit (arbitrary unit). Expanded trace of normalized Rhod-2 intensity during 2 Hz stimulation was presented on the right panel. The downward arrow indicates the point where the expanded trace was extracted. (B) Model-generated mitochondrial Ca2+ transients. Expanded trace during 2 Hz stimulation was also shown on the right panel.
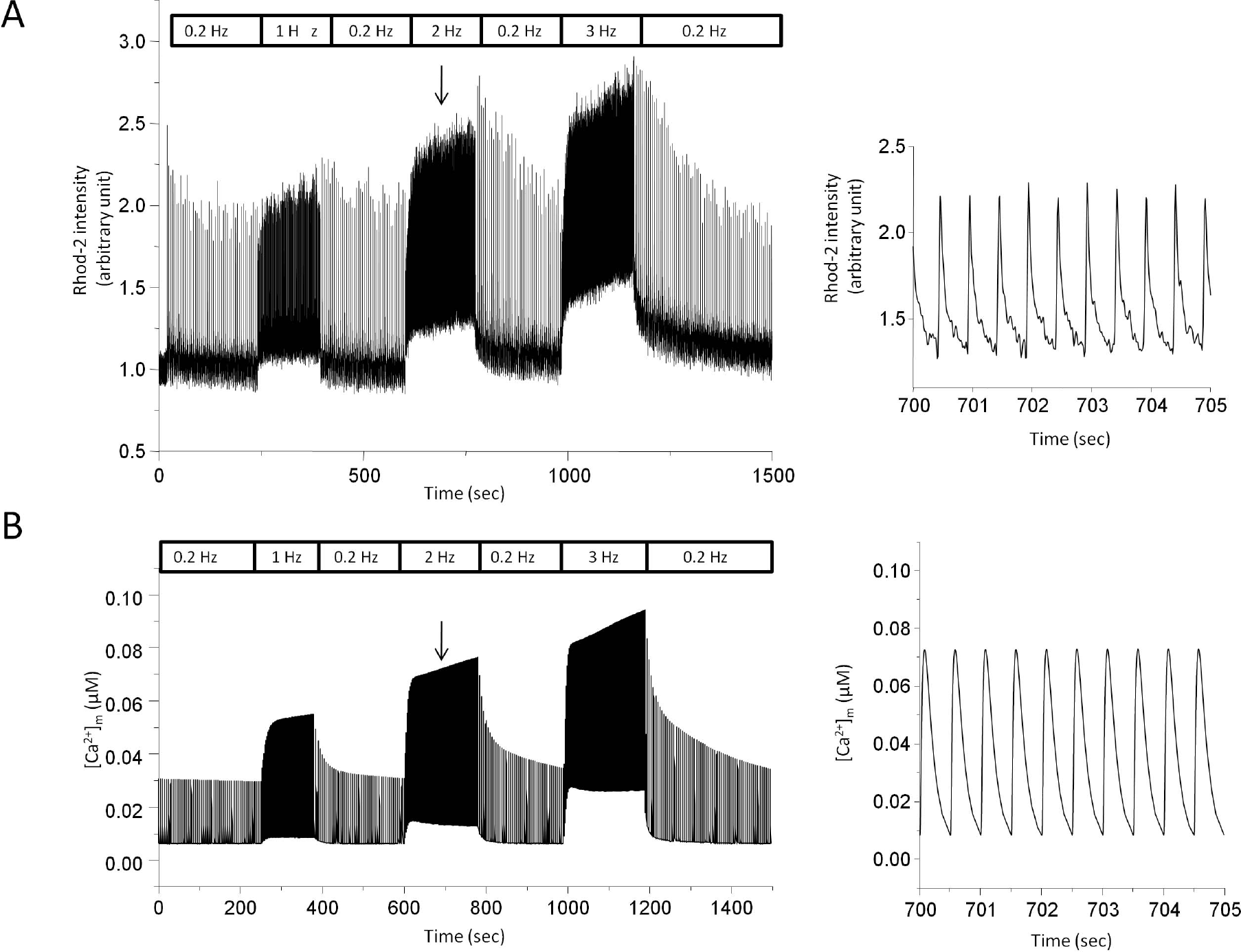
Fig. 5.
The effect of ruthenium red (10μM) on mitochondrial and cytosolic Ca2+ transients. Stimulation rate is 0.2 Hz. (A) Mitochondrial Ca2+ transients during perfusion of ruthenium red. Cells were preincubated with ruthenium red for 5 min before recording. (B) Cytosolic Ca2+ transients during perfusion of ruthenium red.
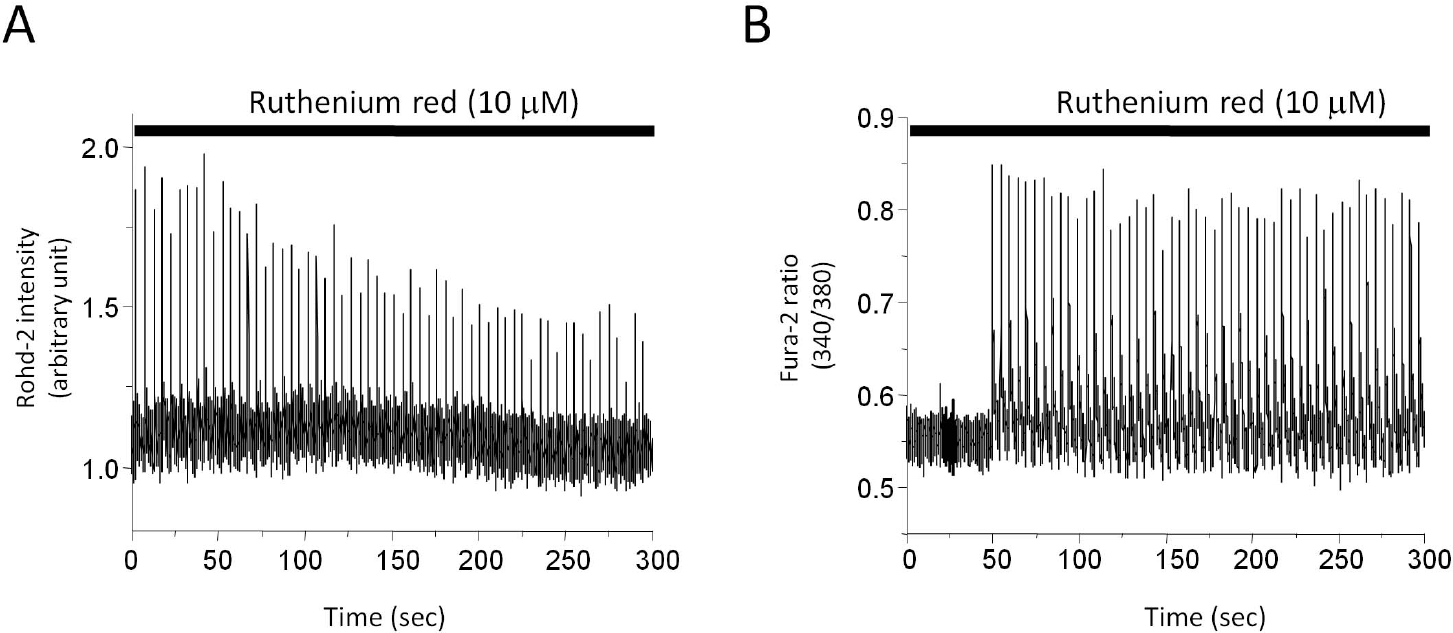
Fig. 6.
The effect of Ca2+ uniporter removal on mitochondrial and cytosolic Ca2+ transients in model simulation. Stimulation rate is 0.2 Hz. Mitochondrial Ca2+ transients with (A) or without (C) Ca2+ uniporter activity. Cytosolic Ca2+ transients with (B) or without (D) Ca2+ uniporter activity.
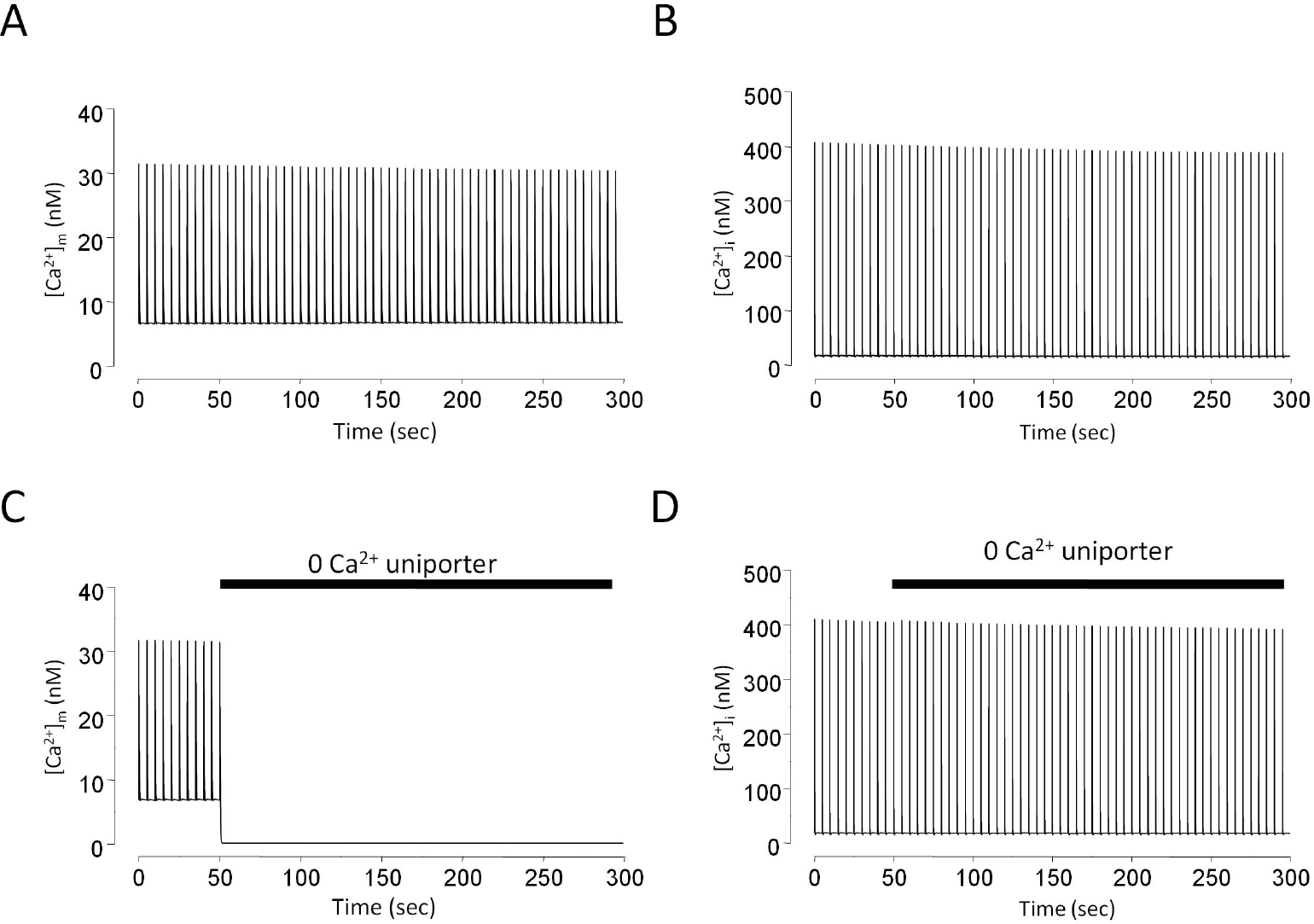
Fig. 7.
The effect of mitochondrial protonophore on cytosolic Ca2+ transients. Stimulation rate is 0.2 Hz. 1μM FCCP eventually abolished Ca2+ transients. Cells became round-up after 5 min perfusion of FCCP. Arrow (A) indicates the point where the expanded trace before FCCP was extracted while arrow (B) indicates the point where expanded trace after FCCP was extracted.
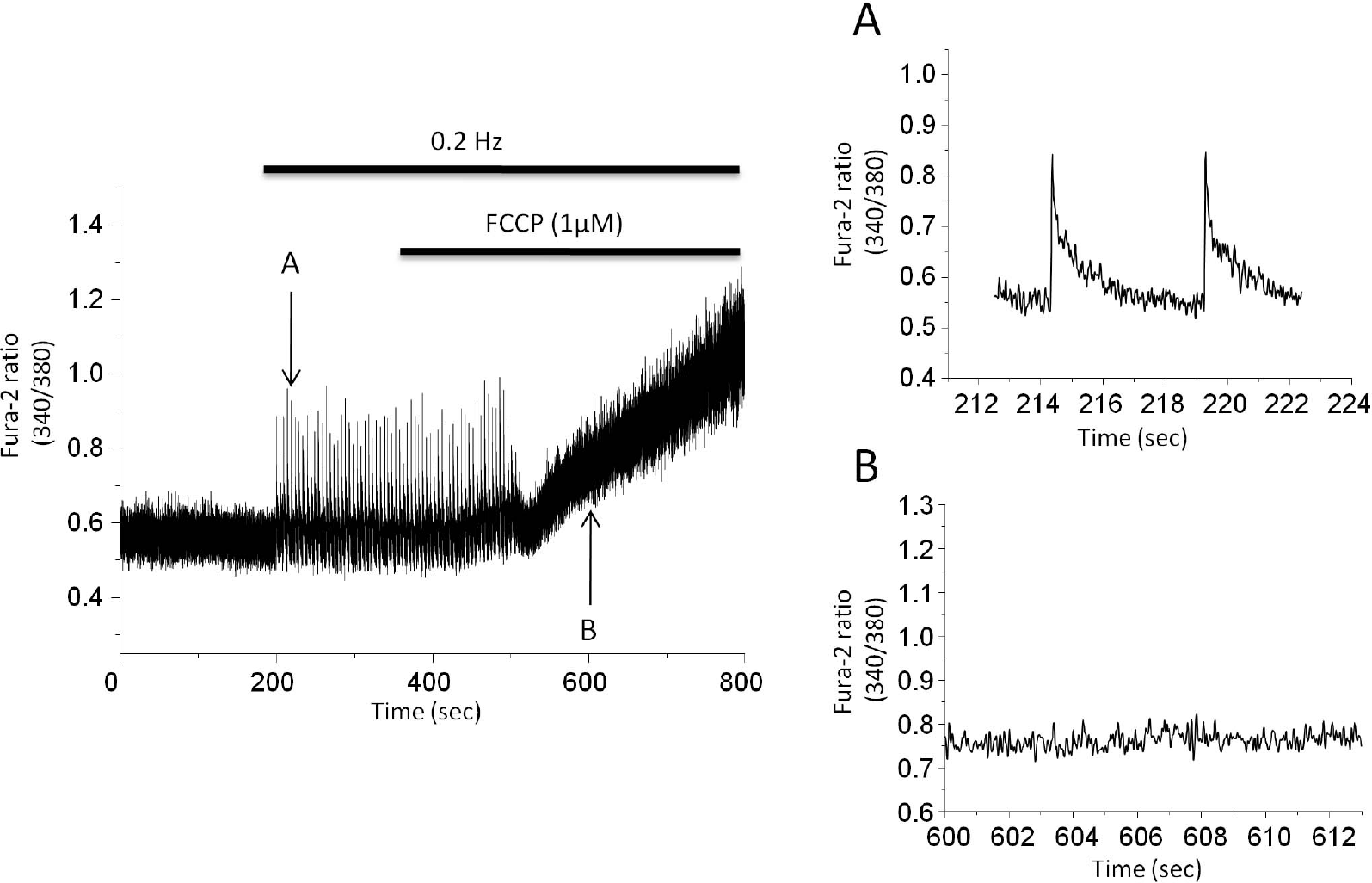
Fig. 8.
Simulation of the effects of mitochondrial protonophore on cytosolic Ca2+ transients and ATP content. Stimulation rate is 0.2 Hz. 106-fold increase in H+ permeability through inner mitochondrial membrane was tested on the model simulation. (A) Change in cytosolic Ca2+ transients during stimulation. (B) Change in cytosolic ATP content during stimulation.
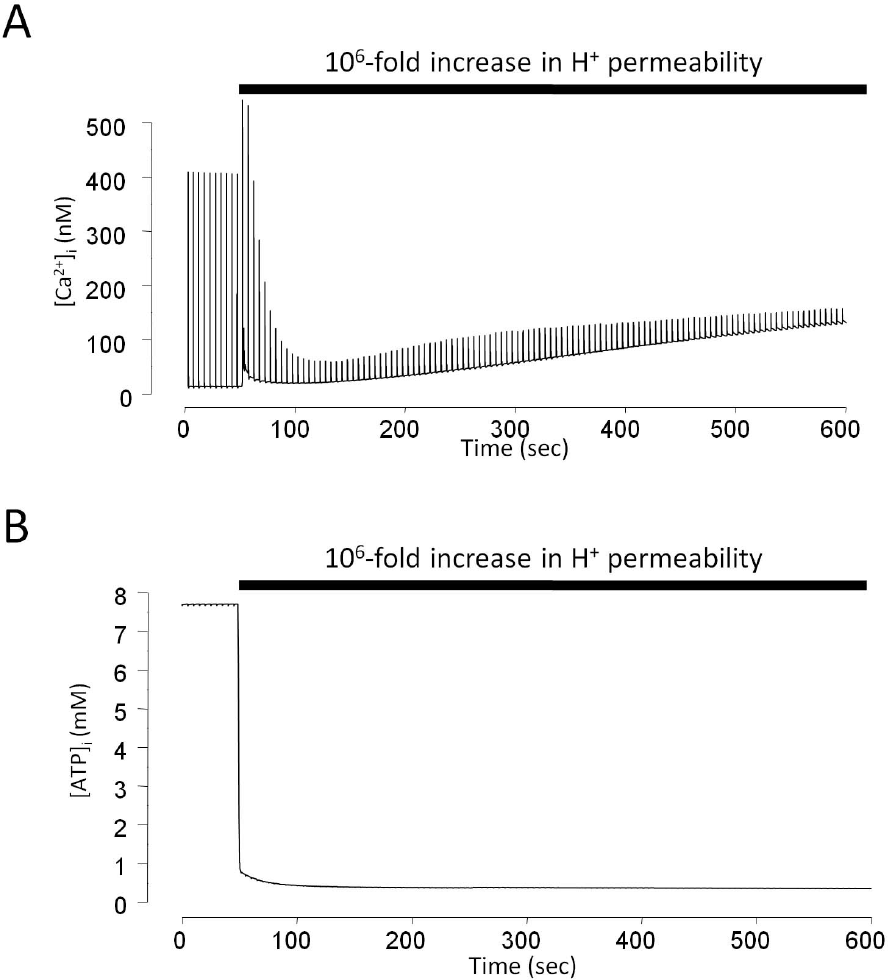
Table 1.
General parameters
Table 2.
Ca2+-binding proteins
Table 3.
Initial values of parameters related with state variables
Table 4.
Initial concentrations of ions and energy metabolites in extramitochondrial space
Table 5.
Definition and initial values of parameters in mitochondrial space